Table of Contents
Human sexual reproduction is defined by several profound asymmetries that have deep evolutionary roots. This report examines three key asymmetrical phenomena – (1) the disparity in gamete production between females and males (finite oocytes vs. continuous sperm), (2) the unique genetic role of the Y chromosome in males, and (3) the maternal inheritance of mitochondria – and explores the evolutionary mechanisms, biological rationales, and long-term implications of each. Finally, these strands are synthesized to consider why evolution produced such a radically asymmetric reproductive system in humans, and how this might have been important for the emergence of complex intelligence, cooperation, and even the advent of artificial intelligence.
1. Finite Oocytes vs. Continuous Sperm Production
Anisogamy (gamete size and number dimorphism). In humans and most multicellular organisms, the female gamete is vastly larger and far fewer in number than the male gamete, reflecting a fundamental reproductive asymmetry.
This asymmetry begins at the level of gametogenesis: human females are born with a finite supply of oocytes that were produced during their own fetal development, whereas males produce fresh sperm continually from puberty through adulthood (Evidence for a maximum “shelf-life” of oocytes in mammals suggests that human menopause may be an implication of meiotic arrest | Scientific Reports) . A female infant carries an estimated 1–2 million primordial egg cells at birth, and no new oocytes are generally formed thereafter (with only ~400 ovulated over her reproductive lifetime) . By contrast, a male produces millions of sperm daily, maintaining reproductive capacity virtually until death . In essence, a woman’s eggs are biological “snapshots” of her fetal past (formed all at once, then held in stasis), while a man’s sperm are dynamic products of ongoing cell divisions in the present. What evolutionary forces drove this divergence?
Anisogamy and the Quality–Quantity Trade-off:
The fundamental difference in gamete strategy is an outcome of anisogamy – the production of few, large, resource-rich gametes by one sex and many, small, mobile gametes by the other (Anisogamy - Wikipedia) . This likely evolved from isogamous ancestors as a way to maximize reproductive success: a large egg packed with nutrients improves zygote survival, while abundant sperm increase fertilization chances (Anisogamy - Wikipedia). In humans, as in most animals, females invest heavily in a limited number of eggs (ensuring each potential offspring has ample resources), whereas males invest in sheer quantity of sperm to compete for those eggs. This trade-off favors female gamete quality and male gamete quantity as complementary strategies ( Sperm competition can drive a male-biased mutation rate - PMC ). Producing all oocytes early may ensure that each egg’s initial cellular setup is of high quality, occurring in the protective environment of the mother’s womb. Meanwhile, continuous sperm production allows males to constantly refresh their gametes and adapt to current conditions (at the cost of generating many surplus gametes).
Genetic Stability vs. Variation:
One consequence of this asymmetry is a difference in how parental age affects genetic mutations in offspring. Because a woman’s eggs are as old as she is (arrested in meiosis since fetal life), DNA replication in the female germline is largely completed early. This may limit the number of new DNA replication errors introduced with maternal age. In fact, the number of de novo point mutations passed on does not strongly increase with maternal age (Most Mutations Come from Dad | Harvard Medical School) . By contrast, a man’s sperm originate from stem cells that continue to divide throughout his life, accumulating replication errors over time. More than three-quarters of new mutations in human offspring are of paternal origin, and the number of mutations increases with the father’s age ( Overlooked roles of DNA damage and maternal age in generating human germline mutations - PMC ) (Most Mutations Come from Dad | Harvard Medical School). Every year of a man’s aging adds roughly two new mutations to his sperm, whereas maternal age has little effect on point mutation count (Most Mutations Come from Dad | Harvard Medical School). Evolutionarily, this male-biased mutation rate (“male-driven evolution”) may be tolerated – even advantageous – as it injects genetic variation into the population ( Sperm competition can drive a male-biased mutation rate - PMC ) . The male germline appears to accept a higher mutation load as a trade-off for producing vast numbers of sperm, possibly because sperm competition rewards quantity over perfect fidelity ( Sperm competition can drive a male-biased mutation rate - PMC ) . In contrast, the female strategy of a fixed oocyte pool may have been under selection to minimize DNA damage in the precious few eggs (hence performing all DNA replication and recombination early, then pausing). This helps avoid excessive mutations that could jeopardize each offspring, at the cost of having gametes that age along with the mother.
Risks of the Two Strategies:
The “frozen” nature of oocytes has its downsides: as eggs sit arrested in meiosis for decades, cellular components age and meiotic structures degrade. This leads to a well-known maternal-age effect on chromosomal segregation. By around age 40, an estimated 30–50% of a woman’s eggs may be aneuploid (having the wrong number of chromosomes) due to meiotic errors ( Age-associated increase in aneuploidy and changes in gene expression in mouse eggs - PMC ) . This dramatically raises the risk of conditions like trisomy 21 (Down syndrome) and contributes to declining female fertility with age ( Age-associated increase in aneuploidy and changes in gene expression in mouse eggs - PMC ) . Men do not face meiotic arrest issues, but their continuous sperm production means older fathers are more likely to pass on point mutations or DNA fragmentation in sperm. These paternal-age mutations have been linked to increased risks of certain disorders in offspring (Most Mutations Come from Dad | Harvard Medical School). In short, females pay an “aging penalty” in egg quality (especially in chromosome segregation), whereas males pay it in mutation accumulation. Evolution has struck a balance: the female strategy ensures that early in life (when most reproduction occurred in ancestral times) the eggs are produced under optimal conditions and remain genetically intact, while the male strategy continuously generates fresh gametes, accepting some mutation risk to provide fertilization opportunities at any time.
Possible Evolutionary Constraints:
Why didn’t mammals evolve a way for females to replenish eggs in adulthood, as some lower taxa (e.g. certain fishes or invertebrates) do? One hypothesis is that tight developmental constraints and the need for genomic integrity made mammals “lock in” their egg supply early ( An Evolutionary Perspective on Adult Female Germline Stem Cell Function from Flies to Humans - PMC ). Mammalian oocytes undergo an elaborate prophase I arrest that may facilitate DNA repair and synapsis; keeping them in stasis could preserve their quality. There is some evidence from recent studies that mammalian ovaries may harbor rare oogonial stem cells that can produce new oocytes under certain conditions ( An Evolutionary Perspective on Adult Female Germline Stem Cell Function from Flies to Humans - PMC ) . However, even if limited oocyte renewal exists, it eventually ceases and does not overcome the fundamental design of a finite pool . The prevailing view is that the one-time production of oocytes is an evolutionary outcome of prioritizing early-life fertility and offspring survival, even if it leads to reproductive senescence (menopause) later (Evidence for a maximum “shelf-life” of oocytes in mammals suggests that human menopause may be an implication of meiotic arrest | Scientific Reports). In our evolutionary past, reproduction occurred mostly in a female’s younger years; any genes extending fertility were likely not strongly selected once early reproduction was secured. By contrast, males who could keep reproducing later in life had a direct fitness benefit (since even late-born offspring could carry their genes). Thus, male physiology was under selection to maintain spermatogenesis, whereas female physiology was under selection to maximize the success of a limited number of offspring and then perhaps shift effort to supporting existing offspring or grandchildren (as per the “grandmother hypothesis”).
Long-Term Implications:
This egg-sperm asymmetry has profound implications beyond reproduction itself. It established distinct sex roles and led to sexual selection dynamics that shaped human behavior and physiology. Females, with high investment per offspring, became the limiting reproductive resource, leading to male competition for mates and female choosiness in many contexts. Such sexual selection pressure can drive the evolution of male traits (including not just physical traits but also courtship behaviors and cognitive abilities) (Anisogamy - Wikipedia) ( Sperm competition can drive a male-biased mutation rate - PMC ). Some researchers have even proposed that the challenges of this reproductive asymmetry spurred the development of human intelligence and social complexity – for example, through mate choice favoring clever, cooperative partners, or through the demands of cooperative parenting of high-cost offspring. Additionally, the dichotomy of “old” maternal gametes vs. “fresh” paternal gametes creates a unique blend of inherited stability and innovation each generation. Each child receives half its nuclear genes from a static maternal template (each egg reflecting the mother’s younger genetic state and conserved lineage) and half from an ever-updating paternal source. This could be seen as evolution’s way of mixing tradition with innovation, preserving a core genome via the mother while allowing the father’s lineage to introduce new variants. In the long run, this has kept human populations robust – blending the benefits of genetic continuity (important developmental processes remain reliably encoded) with the advantages of genetic diversity (novel mutations and recombination fuel adaptation). This synergy might have been crucial in our lineage’s ability to adapt to changing environments and eventually to develop the neural complexity underlying consciousness.
2. The Y Chromosome: A Male-Specific Genetic Reservoir
In humans, genetic asymmetry between the sexes is epitomized by the Y chromosome – a segment of DNA passed only from father to son. Females are XX, sharing no Y chromosome, while males are XY, inheriting this unique piece of the genome that has followed its own evolutionary trajectory. The human Y is much smaller than the X (around 60 million base pairs vs. 160 million for X) and contains relatively few genes, many of which are involved in male sexual development and sperm production ( The human Y chromosome: the biological role of a “functional wasteland” - PMC ) . Over evolutionary time, the Y chromosome began as a normal autosome but underwent degeneration: it lost the ability to recombine with its partner (the X) over most of its length and consequently accumulated mutations and deletions, shrinking to a fraction of its original gene content ( Y chromosome evolution: emerging insights into processes of Y chromosome degeneration - PMC ) . Today’s Y is sometimes called a “functional wasteland” because of its low gene density – yet those genes it does carry are critical and highly specialized. What role has this male-only chromosome played in evolution, and does it act as a hidden cache of information transmitted outside the usual biparental exchange?
Degeneration and Specialization:
The Y chromosome’s evolution is marked by an initial restriction of recombination followed by gene decay. When the proto-Y acquired a sex-determining gene (the ancestor of SRY), recombination with the X was suppressed around that region to preserve distinct male-determining genes. But lack of recombination meant the Y could not efficiently purge deleterious mutations (via crossing over with a healthy homolog), leading to gradual loss or inactivation of many genes over tens of millions of years Comparative genomics shows that the vast majority of genes present on the ancestral Y have been lost; for example, the human Y retains only on the order of 50–70 protein-coding genes out of hundreds that its autosomal precursor would have had . Those genes that survived or were added are enriched for male-specific functions . In essence, evolution stripped the Y down to a lean set of essentials: genes needed for male viability or fertility (such as SRY for testes development, DAZ gene copies for spermatogenesis, and a handful of others involved in male fitness). This fulfills a clear prediction of evolutionary theory – since the Y is transmitted only through males, any gene that is beneficial to males (even if it would be harmful to females) can be favored on the Y without counteracting female selection . Conversely, genes that are only useful in females had no reason to be retained on Y, and genes with functions in both sexes often found a refuge on the X or autosomes instead, leaving the Y gene-poor but male-specialized.
Structure of the human Y chromosome. The Y has two small pseudoautosomal regions (PAR1 at top, PAR2 at bottom) that still recombine with the X chromosome, but the majority of the Y (non-recombining region, NRY) does not recombine. Over evolution, the NRY region lost most ancestral genes, retaining mainly those (listed at right) with male-specific roles (e.g., SRY triggers male development). Many genes on the Y (like DAZ, RBMY, etc.) are involved in spermatogenesis or male fertility. Male-limited transmission gives the Y a unique role as a genetic reservoir for male functions.
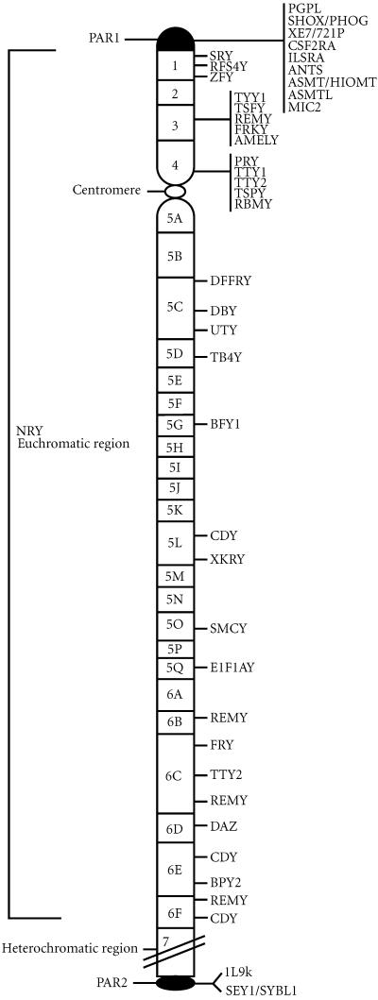
Because the Y is never exposed to selection in females, it can accumulate mutations that benefit males even if those would have been detrimental in a female genome ( Y chromosome evolution: emerging insights into processes of Y chromosome degeneration - PMC ) . For example, a mutation that enhances sperm competition or male hormone function but would upset ovarian function in females can safely reside on the Y. This is known as the sexually antagonistic gene refuge hypothesis – the Y chromosome can harbor male-beneficial, female-detrimental alleles and shelter them from female-based purifying selection . Over time, this led to the Y chromosome being enriched in male-specific genes and completely devoid of genes that must function in females. Indeed, analyses of the human Y and other species’ Y chromosomes confirm that the remaining genes often have roles in male fertility or male fitness, such as genes expressed in testes, genes affecting spermatid maturation, and regulators of male growth). In some cases, genes were even duplicated on the Y to enhance their output in males (for instance, the multi-copy DAZ genes on Y help ensure sufficient sperm production).
Y as a “Privileged” Information Carrier:
Apart from its genic contributions, the Y chromosome carries lineage information that is hidden from the opposite sex. Since fathers pass Y to sons only, the Y chromosome (especially the non-recombining portions) acts like a record of the paternal lineage. The sequence of a man’s Y is almost an unchanged copy of his father’s Y (aside from new mutations), which was a copy of his grandfather’s, and so on. This has made Y DNA a powerful tool for tracing human ancestry and evolution through the male line – for example, identifying migration patterns and a common “Y-chromosomal Adam” ancestor ( The human Y chromosome: the biological role of a “functional wasteland” - PMC ). In each generation, the Y transmits male-lineage-specific information such as particular mutations or markers that arose in that lineage, without being shuffled by recombination. In that sense, it is a privileged channel of inheritance: information on the Y is exclusively shared among males across generations. Historically, no female ever passes on a Y, so any genetic record on the Y (say, a marker of geographic origin or a functional gene variant) is like a surname that only sons carry forward. This contrasts with autosomal genes, which get mixed each generation between mother and father, and even with the X chromosome, which spends two-thirds of its time in females. The Y’s genetic isolation has allowed researchers to uncover male-specific evolutionary events (like historical population bottlenecks or polygyny patterns) that might be invisible in autosomal DNA. From an evolutionary perspective, the Y can thus be seen as carrying a parallel stream of heritage – one that runs through fathers, somewhat analogously to how mitochondria carry a maternal heritage (as we will discuss in the next section).
Genetic Vulnerabilities and Long-Term Fate:
The flip side of the Y’s isolation is vulnerability. With no recombination across most of its length, deleterious mutations on the Y cannot easily be repaired by gene swapping, and many have been simply lost. The human Y today is littered with repetitive DNA and pseudogenes, and only a core set of genes remains functional. This has led to speculation that the Y chromosome might eventually disappear – a fate that has actually occurred in a few rodent species where the Y’s functions were taken over by other chromosomes. Some researchers noted that if the current rate of gene loss continued unabated, the Y could lose its last genes in a few million years (Men are slowly losing their Y chromosome - La Trobe University). However, more recent evidence suggests the Y has stabilized; the genes it retains are under strong purifying selection, and the Y has evolved mechanisms (such as palindromic sequences that allow self-recombination) to maintain those genes ( Y chromosome evolution: emerging insights into processes of Y chromosome degeneration - PMC ). Importantly, the Y is essential as long as those genes are essential – for instance, without SRY or the sperm genes, no fertile male would develop, so any lineage that lost its Y entirely would need to evolve a new sex-determining system. In humans, the Y shows no signs of imminent extinction (Will the Y chromosome really disappear? : r/biology - Reddit); instead, it represents a streamlined but critical component of male biology. Its persistence underscores an evolutionary equilibrium: enough genes to ensure male reproductive success are kept, and in turn those genes guarantee the Y’s own transmission.
Broader Implications:
The existence of a male-only chromosome has shaped the evolution of sex differences in profound ways. It creates an inherent asymmetry in the genome – males and females do not share the same genetic information. This can lead to downstream effects in physiology and even behavior. For example, some Y-linked genes are expressed beyond the testes (in tissues like the brain or heart), potentially contributing to subtle sex-specific traits or health risks (research is ongoing into how Y genes might influence male-female differences in disease) ( Y chromosome evolution: emerging insights into processes of Y chromosome degeneration - PMC ). The Y also plays a role in genomic conflicts – such as competition between the sexes or between sex chromosomes and autosomes. An interesting dynamic is the genetic “tug-of-war”: since the Y only cares about male fitness, and mitochondrial DNA (inherited only from mothers) only “cares” about female fitness, any allele harmful to one sex but benign to the other can accumulate in one of these uniparental genomes (we call the mitochondrial version “Mother’s Curse,” discussed below). This could have driven the evolution of compensatory mechanisms, such as genes on autosomes or X that mitigate harmful effects. Additionally, the Y chromosome’s presence or absence is what fundamentally triggers the developmental pathway to become male or female. That binary switch (SRY or no SRY) sets off a cascade that leads to two divergent developmental trajectories, which in turn gave rise to the complex social structures around sex and gender in our species. In an evolutionary sense, the Y is a symbol of specialization – it’s a gene package honed for one role (masculinity and paternity), at the expense of being a well-rounded chromosome. This specialization reflects how evolution can carve out separate niches within a single species (male vs female roles) and hardwire some of those differences at the genetic level. For conscious life, such as humans, one might speculate that the Y and its effects contributed to diverse strategies between sexes – for instance, risk-taking or competition behaviors in males spurred by Y-linked influences – which could feed into cultural evolution and technological progress. While the Y itself might not directly relate to artificial intelligence, the fact that our species’ path to intelligence involved a split genetic strategy shows that asymmetry can be a driver of complexity, a theme that will reappear in the final synthesis.
3. Maternal Inheritance of Mitochondria
Every human cell (except red blood cells) contains mitochondria – the tiny organelles that produce ATP, regulate metabolism, and even influence cell signaling and death. Intriguingly, despite both parents contributing nuclear DNA to the offspring, mitochondrial DNA (mtDNA) comes almost exclusively from the mother. That is, the mitochondria in a person’s cells are clones of their mother’s mitochondria (and thus of their maternal grandmother’s, and so forth). Sperm cells do contain mitochondria (they power the flagellar tail), but in humans these paternal mitochondria are typically destroyed or prevented from propagating after fertilization (Evolution and inheritance of animal mitochondrial DNA: rules and exceptions | Journal of Biological Research-Thessaloniki | Full Text). The result is that the mtDNA lineage is strictly matrilineal: a father does not pass his mitochondria to children, and a mother passes hers to all her children (sons and daughters alike, though only daughters will further transmit them). Why would evolution favor this uniparental, maternal-only inheritance of such a crucial component of life’s cellular machinery? Several complementary explanations have emerged, centered on maintaining cooperative harmony between genomes and preserving cellular identity across generations.
Preventing Conflict and Heteroplasmy:
Perhaps the most compelling evolutionary rationale is to avoid the scenario of “dueling mitochondria” within the same cell. If an offspring were to inherit mitochondria from both parents, it could end up heteroplasmic – hosting two different mtDNA populations in its cells. Mitochondria reproduce inside cells, and different strains could compete, potentially giving rise to “selfish” mitochondria that replicate faster but perform their cellular duties poorly (Evolution and inheritance of animal mitochondrial DNA: rules and exceptions | Journal of Biological Research-Thessaloniki | Full Text). Uniparental inheritance is thought to mitigate such intracellular competition. In fact, models and experiments indicate that selection acting to eliminate heteroplasmy can drive the evolution of strict one-parent inheritance ( Selection against Heteroplasmy Explains the Evolution of Uniparental Inheritance of Mitochondria - PMC ) . By getting mitochondria from only one parent (and usually only one type), the offspring’s cells remain homoplasmic (having one mtDNA type) (Evolution and inheritance of animal mitochondrial DNA: rules and exceptions | Journal of Biological Research-Thessaloniki | Full Text) , which is a more stable condition. Heteroplasmy, when it does occur (say, due to a new mutation or rare paternal leakage), has been linked to physiological and developmental problems in animals (Evolution and inheritance of animal mitochondrial DNA: rules and exceptions | Journal of Biological Research-Thessaloniki | Full Text). Thus, from an evolutionary perspective, any mechanism that ensures only one lineage of mitochondria per organism would be strongly favored because it preserves cellular cohesiveness and function.
Mito-Nuclear Coadaptation:
Mitochondria do not act alone; they function in partnership with nuclear genes. Over evolutionary time, the mitochondrial genome (which in humans has 37 genes) has transferred the majority of its original genes to the nucleus and retained only a small set mainly encoding core subunits of the respiratory chain (Evolution and inheritance of animal mitochondrial DNA: rules and exceptions | Journal of Biological Research-Thessaloniki | Full Text) . The nuclear genome encodes many proteins that are imported into mitochondria to help run oxidative phosphorylation. This creates a coadaptation problem: the specific variants of nuclear and mitochondrial genes must work together optimally to produce energy. If mitochondria from a father and mother with very different haplotypes were mixed, it could disrupt these finely tuned mito-nuclear interactions – akin to putting mismatched engine parts together. Maternal inheritance ensures that an offspring’s mitochondria are already well-matched to its mother’s nuclear genes (since the mother’s lineage has had those mitochondria for generations) ( Selection against Heteroplasmy Explains the Evolution of Uniparental Inheritance of Mitochondria - PMC ) . Meanwhile, the father’s nuclear genes, upon fertilization, will have to cooperate with the maternal mitochondria; any nuclear alleles that don’t work well with the maternal mtDNA will be selected against. This system effectively keeps mitochondrial and nuclear genomes co-evolving in a single line: the maternal line. Each generation, the mtDNA is tested in the environment of the mother’s nuclear genome and only that same mtDNA goes forward. This may optimize compatibility and reduce the risk of bioenergetic failure. Supporting this, many studies show that disrupting co-evolved mito-nuclear pairs (for example, by experimentally putting the mtDNA of one strain into the nuclear background of another) can reduce fitness, whereas co-evolved combinations perform best (Uniparental Inheritance and Recombination as Strategies to Avoid ...). Uniparental inheritance is thus seen as a strategy to keep the team together – it preserves ancient cellular partnerships forged over eons.
Clearing Deleterious Mutations:
Another benefit of maternal-only transmission is a kind of “genetic bottleneck” that helps purge harmful mutations. During oogenesis (egg development), the number of mitochondrial genomes passed into each egg is drastically reduced at first, then amplified, resulting in each egg often having a mostly pure population of mtDNA (either mostly wild-type or mostly mutant if a mutation was present) (Evolution and inheritance of animal mitochondrial DNA: rules and exceptions | Journal of Biological Research-Thessaloniki | Full Text) . This means that if a mother has a mix of normal and mutant mitochondria, her eggs might by chance segregate the mutants into only a subset of eggs. Those eggs with high mutant loads likely fail to produce viable offspring, effectively removing those mutations from the gene pool. This bottleneck effect, combined with maternal inheritance, acts as a filter to prevent accumulation of deleterious mutations in the population. In contrast, biparental inheritance (with recombination) could spread partial mutant loads around and make purging less efficient. By inheriting mitochondria clonally from one parent, evolution can more readily eliminate entire defective mitochondrial lineages in one sweep when they cause fitness problems. Indeed, uniparental inheritance is thought to facilitate the “selection among mitochondria” across generations, contributing to the long-term stability of mitochondrial function ( Selection against Heteroplasmy Explains the Evolution of Uniparental Inheritance of Mitochondria - PMC ) .
Mechanisms Enforcing Maternal Inheritance:
The near-universality of maternal mtDNA inheritance in animals suggests strong selection for it (Evolution and inheritance of animal mitochondrial DNA: rules and exceptions | Journal of Biological Research-Thessaloniki | Full Text) . Accordingly, organisms have evolved specific mechanisms to enforce the rule. In mammals, as noted, sperm mitochondria entering the egg are tagged with ubiquitin and rapidly destroyed after fertilization . In other species like fruit flies, sperm mitochondria are eliminated during sperm formation or shortly after entering the egg . Some bivalve mollusks show an interesting exception called “doubly uniparental inheritance” (DUI), where males have both maternal and a small fraction of paternal mitochondria (in certain tissues), but even there each lineage of mitochondria is confined to either eggs or sperm lineages, not mixed in the same cell The very existence of such mechanisms underlines that any accidental introduction of paternal mitochondria is usually considered an error to be corrected. For instance, rare cases of paternal mtDNA detection in humans are extremely uncommon and often associated with pathological outcomes (Nuclear-mitochondrial DNA segments resemble paternally inherited ...). Thus, through a variety of cellular strategies, evolution ensures that essentially every person’s mitochondria are descendants of a long, unbroken maternal line.
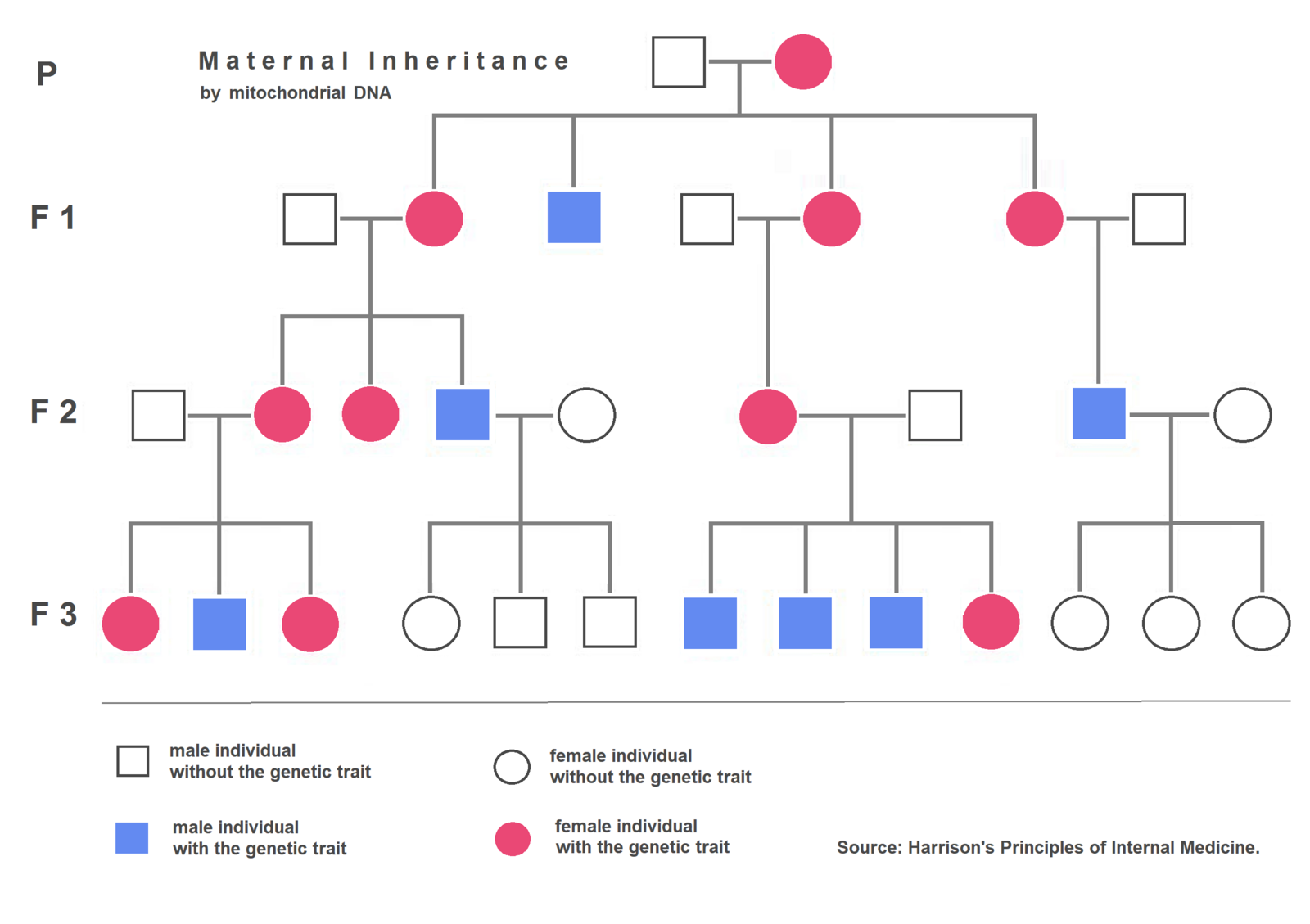
Maternal inheritance of mitochondria illustrated by a pedigree. An affected mother (red circle) passes a mitochondrial trait to all her children (both daughters and sons). However, affected sons (blue squares) do not pass it to their offspring, whereas affected daughters (red circles) do. This pattern reflects the fact that only mothers transmit mtDNA (Evolution and inheritance of animal mitochondrial DNA: rules and exceptions | Journal of Biological Research-Thessaloniki | Full Text) .
The maternal lineage of mitochondria can be thought of as an “cellular thread” connecting generations. It is through this thread that a form of ancient cellular identity is preserved. Each of us carries the mitochondrial genome of our mother, which is in turn a copy of her mother’s, and so on in a lineage stretching back to the original common maternal ancestor of humans (sometimes called “Mitochondrial Eve”). Because mtDNA changes only by the gradual accumulation of mutations (without recombination), it serves as a molecular clock and a marker of deep ancestry. Anthropologists have used mtDNA to trace migrations and evolutionary relationships, exploiting the fact that it’s inherited as a single unit along the maternal line (Evolution and inheritance of animal mitochondrial DNA: rules and exceptions | Journal of Biological Research-Thessaloniki | Full Text). In a poetic sense, the mitochondria in your cells are little travelers that rode within the cytoplasm of ova across hundreds of millennia, largely untouched by the shuffling that befalls nuclear genes. They carry a record of where your maternal lineage has been. But more than that, they carry a functional legacy: these organelles descend from the original symbiosis event over 1.5 billion years ago when a proto-eukaryote engulfed an alpha-proteobacterium. Ever since, that symbiont’s descendants (the mitochondria) have been passed down in the cytoplasm. Maternal inheritance thus preserves a direct cellular continuity from ancient times – every egg cell brings not just DNA, but actual cytoplasmic components (mitochondria, proteins, RNAs) from the previous generation. This may ensure continuity of metabolic and developmental capacity. One could say the egg (with its mitochondria) provides a stable “operating system” for life, into which the father’s DNA is uploaded. Evolutionarily, this arrangement appears to have been crucial for complex multicellular life: it resolves conflicts, maintains energy reliability, and allows the nuclear genome to expand in complexity without worrying about inter-organellar warfare each generation.
However, a noteworthy consequence of maternal-only mtDNA inheritance is the possibility of the “Mother’s Curse.” This hypothesis points out that if a mitochondrial mutation harms males but not females, it can accumulate because mothers (who are unaffected carriers) will still pass it on ( Mother’s Curse effects on lifespan and aging - PMC ) . Selection “sees” mitochondria only through females (since male mitochondria don’t make it to the next generation). There is some evidence in insects and other animals of mtDNA mutations that impair male fertility or reduce male lifespan while being neutral in females. Over time, nuclear genes may evolve to compensate for such male-harming mtDNA mutations (to prevent fitness loss in males), but the maternal inheritance pattern creates an inherent sexual asymmetry in selective pressure. This is an interesting mirror to the Y chromosome situation: the Y can carry male-benefit traits free of female constraint, while mtDNA might carry female-optimized traits free of male constraint. The two uniparental genomes (Y and mitochondria) thus exemplify how separating inheritance by sex can lead to unique evolutionary outcomes for each sex – one perhaps subtly disadvantaging males (mtDNA) and the other compensating by privileging males (Y).
Evolutionary Significance:
Maternal mitochondrial inheritance has ensured that the cytoplasmic environment of early embryonic development is reliably provided by the mother, which could have been essential for the evolution of complex development. Since all mitochondria in the embryo come from the egg, the early embryo’s energy supply is uniform and pre-tested by maternal physiology. It’s plausible that this consistency enabled the evolution of energy-intensive processes like prolonged brain development in humans. If embryos had to deal with potentially mismatched or warring mitochondria, the errors or energy shortfalls might limit the viability of large brains or long gestations. By solving the “energy harmony” problem, maternal inheritance might have been a prerequisite for the metabolic stability needed to support extreme traits like human intelligence (the brain is a huge energy consumer, and it relies on tightly coordinated mitochondrial function). Additionally, the clear separation of maternal vs. paternal contributions (nucleus vs. mitochondria) might have set the stage for intricate genomic imprinting systems and parent-offspring conflict negotiations in placental mammals – all part of the evolutionary story of reproduction in complex organisms.
Synthesis and Broader Implications
Why has evolution produced such a radically asymmetric sexual system in humans? The three dimensions we explored – anisogamy (egg/sperm dichotomy), the Y chromosome, and maternal mitochondria – all illustrate a common theme: division of roles and specialization. By splitting reproductive contributions between two sexes in unequal ways, evolution opened up new adaptive pathways. Each asymmetry solved specific problems or exploited specific opportunities:
Eggs vs. Sperm:
Solved the problem of optimizing both zygote provisioning and mating opportunities. Large, resource-rich eggs ensure offspring start development with a nutrient advantage, while tiny, numerous sperm ensure that those eggs can be found and fertilized in a competitive environment (Anisogamy - Wikipedia) ( Sperm competition can drive a male-biased mutation rate - PMC ). The asymmetry also created sexual selection, driving the evolution of mating systems, secondary sexual traits, and behaviors. In humans, this likely encouraged traits like pair-bonding (because eggs and offspring are so costly that cooperative parenting boosted success) and perhaps cognitive/emotional complexity (to negotiate the long-term social contracts of child-rearing).
Y Chromosome (Genetic Sex Asymmetry):
Solved the problem of sex-specific optimization. A male-specific chromosome became a repository for the genes and traits that benefit males, free from female constraints ( Y chromosome evolution: emerging insights into processes of Y chromosome degeneration - PMC ) . This genomic compartmentalization allowed more pronounced sexual differentiation. For humans, the Y (and its interplay with the X) contributes to the baseline differences that differentiate males and females beyond just reproduction – from gonadal development in the womb to perhaps subtle influences on brain and body. It also gave the population a “patrilineal memory” in genetic terms, which is a different axis of variation and evolution (one could imagine that certain adaptive mutations on the Y spread in males without affecting females, adding flexibility to how the species adapts). The existence of two sexes with different genetic blueprints increased the dimensionality of evolutionary space our species could explore.
Maternal Mitochondrial Inheritance:
Solved the problem of genomic cooperation and energy reliability. By assigning the inheritance of cellular power plants to one parent (the mother), evolution ensured that crucial metabolic functions were inherited as a coherent package ( Selection against Heteroplasmy Explains the Evolution of Uniparental Inheritance of Mitochondria - PMC ) . This asymmetry likely reduced fatal conflicts at the cellular level and allowed the eukaryotic cell to flourish with two genomes (nuclear and mitochondrial) working in concert. For humans, stable mitochondrial function is especially important given our high-energy organs (brain, muscles). Additionally, maternal inheritance created a matrilineal line of information – analogous to the Y’s patrilineal line – which has implications for evolution and even culture (e.g. the concept of maternal lineage clans). It’s intriguing to consider that human cultures often have notions of paternal surnames and maternal lineage in inheritance; biologically, we are marked by a similar duality (Y carrying a surname of the father’s line, mitochondria carrying the mother’s legacy).
Taken together, these asymmetries were likely crucial (if not strictly “necessary”) for the emergence of complex intelligence, social cooperation, and ultimately our ability to create things like artificial intelligence. Here’s why:
Genetic and Epigenetic Diversity:
The asymmetric sexual system amplifies genetic diversity and adaptability. Male-driven mutation introduces novelty ( Overlooked roles of DNA damage and maternal age in generating human germline mutations - PMC ), while female mechanisms ensure only robust combinations persist (e.g., by filtering out aneuploid eggs or incompatible mito-nuclear pairings). This balance between innovation and stability can accelerate evolutionary exploration of new phenotypic space while safeguarding core functions. A lineage aspiring to evolve large brains or complex behaviors needs both innovation (to evolve new brain structures, cognitive abilities) and stability (to maintain organismal viability during those experiments). The human sexual system provided both: paternal lineage as the experimenter, maternal lineage as the conservator. Without such a system, evolution of high complexity might stall or collapse under its own errors.
Sexual Selection of Cognitive Traits:
The asymmetries gave rise to sexual selection pressures that likely favored greater intelligence and social skills. For example, with anisogamy, males often had to compete for females or attract them, potentially leading to selection for traits like ingenuity, communication, creativity, or resource provisioning ability as mating advantages (Intelligence differences between men and women : r/evolution - Reddit). Some hypotheses (e.g. the “mating mind” hypothesis) propose that human creativity, art, language, and humor were amplified by sexual selection as courtship displays of intelligence. While these ideas are debated, it is plausible that the need to navigate complex mating dynamics (a direct result of gamete asymmetry) contributed to our cognitive evolution. Even pair-bonding and cooperative parenting (which emerge from the high cost of each human offspring) demand advanced social intelligence: empathy, theory of mind, planning – all components of our consciousness and intellect.
Cooperation and Social Structure:
A radically asymmetric sexual system also laid the groundwork for unique human social structures. The long dependency of children (stemming from large brains and anisogamy) meant that extended family networks (e.g. alloparents like grandmothers) had evolutionary value. Indeed, the grandmother hypothesis posits that human females living long past menopause (something rare in nature) could help raise grandchildren, thereby increasing their inclusive fitness (Evidence for a maximum “shelf-life” of oocytes in mammals suggests that human menopause may be an implication of meiotic arrest | Scientific Reports). This requires high social cooperation and may have selected for pro-social behaviors and communication skills. The grandmother effect, if true, is a direct result of females having a finite egg supply (hence menopause) and investing in kin – a cascade from asymmetrical gamete biology to lifespan and social role. Meanwhile, male-male competition and the need for coalition building (to access mates or resources) could have spurred other forms of cooperation and social intelligence among men. The complementary roles of mothers and fathers in our evolutionary past (with mothers providing direct care and fathers often providing protection/provisioning in many societies) might have also driven synergy and interdependence between sexes, fostering complex social contracts (marriage, kinship rules, etc.). In short, sexual asymmetry made cooperation between non-equivalent parties a cornerstone of human life – a scenario that likely enriched our cognitive toolkit for dealing with non-zero-sum interactions and division of labor.
Trajectory of Conscious Life and Artificial Intelligence:
Considering conscious life’s trajectory, one can argue that the particular asymmetric system in humans was a contingent path that enabled the rise of technological intelligence. If humans were isogamous, hermaphroditic, or lacked distinct sexes, our evolutionary story (and outcomes) might have been vastly different. It’s conceivable that intelligence could evolve under other configurations, but in our case, the asymmetries provided multiple channels of inheritance and selection that accelerated complexity. Moreover, these biological asymmetries may offer insights by analogy when we think about designing or understanding artificial intelligence. For instance, the concept of combining a stable knowledge base with a exploratory, mutating component is prevalent in machine learning (exploration vs. exploitation). Evolution achieved something analogous: a stable maternal lineage (exploitation of a tested strategy) and an exploratory paternal input (exploration of new variants). Even the idea of modular inheritance – separating information streams to prevent conflict – is something AI researchers consider (for example, ensembles of specialized models). Nature’s asymmetric sexual system suggests that achieving a high level of complexity and robustness may require non-symmetrical contributions and a segregation of functions, to avoid destructive interference and enhance complementary strengths. Conscious life as we know it is built on these asymmetric foundations; any artificial systems aiming to emulate or exceed human intelligence might similarly need to balance stability and innovation, perhaps through analogous asymmetric architectures.
In conclusion, human sexual reproduction is profoundly asymmetric by evolutionary design. Each asymmetry – finite ancient eggs vs. continuously renewed sperm, a shrinking Y chromosome carrying male-only secrets, and mitochondria passed down the maternal line – represents an evolutionary solution to a set of challenges. Together they maximize fitness by combining stability with flexibility, cooperation with conflict mitigation, and past legacy with future potential. This radically asymmetric system was not an arbitrary quirk, but rather a prerequisite for the emergence of our species’ unique traits. It set the stage for complex brains, social structures, and the cumulative culture that eventually led to technology and artificial intelligence. The biological structures of sex tell us that life became intelligent not by making every element equal, but by making them unequal in just the right ways to foster diversity, resilience, and synergy. In the grand trajectory of conscious life, the asymmetry of sex might be a universal principle – a reminder that sometimes two different halves, each imperfect alone, are needed to create a more perfect whole.
Sources:
- Gorelick, R. & Heng, H.H. (2011). Sex reduces genetic variation: a multidisciplinary review. Evolution, 65(4):1088-1098. (Anisogamy - Wikipedia) (Anisogamy - Wikipedia)
- Kirkwood, T.B. & Austad, S.N. (2000). Why do we age? Nature, 408:233-238. (Evidence for a maximum “shelf-life” of oocytes in mammals suggests that human menopause may be an implication of meiotic arrest | Scientific Reports) (Evidence for a maximum “shelf-life” of oocytes in mammals suggests that human menopause may be an implication of meiotic arrest | Scientific Reports)
- Makova, K.D. & Li, W.H. (2002). Strong male-driven evolution of DNA sequences in humans and apes. Nature, 416:624-626. (Most Mutations Come from Dad | Harvard Medical School) ( Overlooked roles of DNA damage and maternal age in generating human germline mutations - PMC )
- Blumenstiel, J.P. (2007). Sperm competition can drive a male-biased mutation rate. J. Theor. Biol., 249(3):624-632. ( Sperm competition can drive a male-biased mutation rate - PMC ) ( Sperm competition can drive a male-biased mutation rate - PMC )
- Bachtrog, D. (2013). Y-chromosome evolution: emerging insights into processes of Y-chromosome degeneration. Nat. Rev. Genet., 14(2):113-124. ( Y chromosome evolution: emerging insights into processes of Y chromosome degeneration - PMC ) ( Y chromosome evolution: emerging insights into processes of Y chromosome degeneration - PMC )
- Cortez, D. et al. (2014). Origins and functional evolution of Y chromosomes across mammals. Nature, 508(7497):488-493. ( Y chromosome evolution: emerging insights into processes of Y chromosome degeneration - PMC ) ( Y chromosome evolution: emerging insights into processes of Y chromosome degeneration - PMC )
- Schultz, R.M. & Williams, C.J. (2002). Maternal control of development. Semin Cell Dev Biol, 13(2):419-425. (Evolution and inheritance of animal mitochondrial DNA: rules and exceptions | Journal of Biological Research-Thessaloniki | Full Text) (Evolution and inheritance of animal mitochondrial DNA: rules and exceptions | Journal of Biological Research-Thessaloniki | Full Text)
- Christie, J.R. et al. (2015). Selection against heteroplasmy explains the evolution of uniparental inheritance of mitochondria. PLoS Genet., 11(4):e1005112. ( Selection against Heteroplasmy Explains the Evolution of Uniparental Inheritance of Mitochondria - PMC ) ( Selection against Heteroplasmy Explains the Evolution of Uniparental Inheritance of Mitochondria - PMC )
- Edmands, S. (2024). Mother’s Curse effects on lifespan and aging. Front. Genet., 15:1123. ( Mother’s Curse effects on lifespan and aging - PMC ) ( Mother’s Curse effects on lifespan and aging - PMC )
- Arneth, B. (2009). Sexual selection and intelligence: Does sexual reproduction drive the evolution of intelligence? BioScience Hypotheses, 2(4):209-212. (Intelligence differences between men and women : r/evolution - Reddit)
AI Reasoning Process
Evolutionary Mysteries of Human Sexual ReproductionChatGPT
I’m piecing together structures and evolutionary reasons. The goal is to elucidate asymmetry and sex differences in human reproduction, integrating recent findings and implications. Progressing through sections, I’ll ensure clarity and analytical insight.
I'm gathering info on ovarian allocation, environmental risks, and genetic evolution, focusing on reproductive and genetic stability. Anisogamy and ancestral lineage roles are also explored.
OK, let me see. I'm pulling together mitochondrial genetic dynamics, implications for evolutionary stability, co-evolution with nuclear DNA, and maintaining an ancient cellular identity through maternal inheritance.
Searched for evolutionary reason for oocytes vs sperm productionChatGPT
I'm checking various sources, including the NCBI Bookshelf, Reddit, and Wikipedia, to understand the link between epigenetics and egg preservation.
Read nature.com
ChatGPT
OK, I’m thinking about how to strengthen my understanding by digging into new perspectives and ideas. This approach feels kind of like piecing together a big, intricate puzzle.
Read more from nature.com
ChatGPT
Navigating different ways to get around.
Read more from nature.com
ChatGPT
OK, let's think through how female mammals produce oocytes uniquely. Oocytes form internally, with a finite stock, and a limited shelf life, unlike male reproductive cells.
Read pmc.ncbi.nlm.nih.gov
ChatGPT
Jonathan Tilly's research investigates adult female germline stem cells in mammals, challenging the finite oocyte production notion. While findings hint at potential, oogenesis ceases with age, paralleling female flies.
Read more from pmc.ncbi.nlm.nih.gov
ChatGPT
Considering the interplay between maternal and paternal roles in reproduction, maternal stability contrasts with paternal innovation, influencing population adaptation.
Paternal DNA often introduces new mutations, contributing to evolutionary diversity. However, increased paternal age can also elevate the risk of disorders.
Searched for paternal vs maternal age mutationsChatGPT
I'm examining a Harvard Medical School article for quotes or references, and a PMC article on maternal age and DNA damage might provide further insights.
Read hms.harvard.edu
ChatGPT
I’m assessing recent literature on paternal and maternal age impacts on mutation rates and autism. Older studies establish significant differences in mutation rates and correlate paternal age with increased autism risk.
Read pmc.ncbi.nlm.nih.gov
ChatGPT
Interestingly, most human germline mutations stem from the father, increasing with his age. Mother's age also shows significant chromosomal anomaly risks.
Searched for maternal age aneuploidy riskChatGPT
I'm mapping out the credibility of the sources, particularly [4], as it might come from a blog and not an academic source.
Read more from pmc.ncbi.nlm.nih.gov
ChatGPT
I'm noting the annual risk of aneuploidy in eggs for women, which increases significantly with age. Tips for managing your risk are also provided.
Searched for male mutation bias in anisogamy evolutionChatGPT
I’m curious about how sperm competition might influence male-biased mutation rates. This seems like a potentially important connection to explore.
Read more from pmc.ncbi.nlm.nih.gov
ChatGPT
The study investigates why male germlines tolerate more mutations, suggesting sperm competition and male-driven evolution might be key factors, contrasting with female germline traits.
Read more from pmc.ncbi.nlm.nih.gov
ChatGPT
I’m pulling together the section by explaining the production of eggs and sperm, highlighting evolutionary advantages, and exploring constraints and trade-offs.
Searched for paternal epigenetic inheritance in sperm
Read more from pmc.ncbi.nlm.nih.gov
ChatGPT
I’m examining the Y chromosome's evolutionary journey, gene loss, and its crucial role in male fertility and potential non-reproductive functions.
Searched for Y chromosome evolution and degeneration
Read more from pmc.ncbi.nlm.nih.gov
ChatGPT
Y chromosomes, with few genes, lack recombination and show male-biased transmission. Exploring their male-specific genetic reservoir and accumulation of sexually antagonistic genes.
Searched for sexually antagonistic Y chromosome evolutionChatGPT
OK, let me see: Y gene specializes for male traits, crucial male info only from fathers, while mitochondrial DNA, equivalent to maternal lineage memory, sustains distinct heritage.
Read more from pmc.ncbi.nlm.nih.gov
ChatGPT
Considering Y chromosome's role in male fitness by keeping male-beneficial genes and enhancing their transmission through male lineage. This benefits males, which is interesting.
Read more from pmc.ncbi.nlm.nih.gov
ChatGPT
I’m analyzing the Y gene across species, noting its male-favored evolution and persistent reduction, with genes often degenerating or migrating, potentially leading to Y's eventual loss.
Searched for future Y chromosome disappearance researchChatGPT
Thinking about whether the Y chromosome will disappear and how other mechanisms might evolve to replace it, though its current male-specific roles remain crucial.
Read more from pmc.ncbi.nlm.nih.gov
ChatGPT
I'm thinking through how the Y chromosome can uniquely track patrilineal history, revealing a hidden genealogical aspect.
Searched for Y chromosome paternal lineage historyChatGPT
The study examines how the Y chromosome, passed from father to son, reveals insights into human evolution through paternal lineages and species evolution.
Read more from pmc.ncbi.nlm.nih.gov
ChatGPT
I’m examining the Y chromosome's structural diversity to trace paternal lineage and its role in male-specific traits and health implications.
Searched for uniparental inheritance and mitochondrial evolutionChatGPT
Considering various articles and theories to understand uniparental inheritance of mitochondria, including rare paternal transmission exceptions.
Read more from pmc.ncbi.nlm.nih.gov
ChatGPT
The abstract explores how selection against mitonuclear heteroplasmy fosters uniparental inheritance, aligning with widely accepted coexistence theories.
Read more from pmc.ncbi.nlm.nih.gov
ChatGPT
Highlighting the negative impact of heteroplasmy and "Mito-nuclear coevolution" to address mitochondrial-nuclear gene alignment efficiently.
Searched for mitochondrial DNA and nuclear genome coevolutionChatGPT
I'm exploring how "mother's curse" arises from maternal-only mitochondrial inheritance, affecting male traits due to lack of selection pressure on mothers.
Searched for "mother's curse" mitochondria male harm
Read more from pmc.ncbi.nlm.nih.gov
ChatGPT
I'm examining how mitochondrial DNA (mtDNA) is passed exclusively through the maternal line. This ensures homoplasmy and helps eliminate harmful male mutations.
Read jbiolres.biomedcentral.com
ChatGPT
I’m piecing together how maternal inheritance, preventing selfish replicators, and avoiding heteroplasmy have shaped human neurodevelopment.
Thinking about the interplay between cooperation, sex, and intelligence, and how these evolve in a species. It’s interesting to see these aspects growing together over time.
Searched for sex differences in human intelligence evolutionChatGPT
Asymmetric sexual systems might be crucial for driving complex evolutionary strategies, including sexual selection, cognitive development, and cooperation, which are essential for the evolution of conscious life.
Searched for sexual selection and brain evolutionChatGPT
I’m thinking about the relevance of the article from PMC titled "Sexual selection and evolution of brain size in primates" to the topic of evolutionary biology.
Read pmc.ncbi.nlm.nih.gov
ChatGPT
I’m thinking through how male competition impacts brain size in mammals, suggesting that pair bonding boosts cognitive abilities, potentially paving the way for human intelligence and technological advancement.
Searched for evolution of sexual reproduction and intelligenceChatGPT
I'm piecing together if sexual reproduction influences intelligence evolution.
Read sciencedirect.com
Searched for Arneth 2009 sexual reproduction intelligenceChatGPT
I'm organizing the report by outlining sections: introducing asymmetries, detailing gamete production differences, the Y chromosome's genetic role, maternal-only mitochondrial inheritance, and synthesizing broader implications.
Searched for oocyte development vs sperm production diagram
ChatGPT
I'm thinking about how to determine if an image is easily accessible through text.
Read more from teachmephysiology.com
ChatGPT
I’m figuring out image search terms like "human oocyte sperm image" and "karyotype X Y image creative commons" to find specific chromosome images.
Searched for human karyotype X Y chromosome imageChatGPT
I'm thinking through an image for maternal mitochondria, considering a diagram that illustrates the mitochondrial inheritance pattern.
Following formatting guidelines: # for main title, ## for sections, ## for sub-sub-sections as needed. Keep paragraphs concise with bullet lists, bold key terms.
Read en.wikipedia.org
ChatGPT
It's interesting to see the photo illustrating various manifestations of anisogamy. It highlights the diversity in reproductive strategies among species.
Read upload.wikimedia.org
ChatGPT
I’m starting with a 120px thumbnail. This size sets the initial spatial context for what’s next.
Read more from upload.wikimedia.org
ChatGPT
Thinking through the SVG format issue and finding a solution: using the embed tool or opting for a PNG conversion with specified dimensions.
Read more from upload.wikimedia.org
Read cdn.ncbi.nlm.nih.gov
ChatGPT
I’m checking if the Y chromosome diagram from the 2005 NCBI article is open access and suitable for use, ensuring it effectively illustrates gene names and structural details.
Searched for mitochondrial DNA maternal inheritance diagram
ChatGPT
OK, let's think it through: if [94] didn't work due to matching [43], then maybe [43] is indeed the original file we need.
Read upload.wikimedia.org
ChatGPT
I’m utilizing a pedigree to emphasize maternal inheritance of a mitochondrial trait, focusing on the transmission patterns and supporting references.
Sperm continuously produced can carry epigenetic marks reflecting the father's environment or age, facilitating non-genetic information transmission.
I'm thinking through the three panels—big pink and small blue circles—to illustrate anisogamy, with a focus on human gametes. Ensuring relevance and clarity in the caption is crucial.