Table of Contents
Aim
- What we currently understand about morphogenesis.
- The revolutionary insights emerging from Levin’s bioelectrical and cognitive model.
- Open questions and uncharted paths that might hold the key to life’s most persistent mysteries — from regeneration to embodied intelligence to consciousness itself.
Introduction
Morphogenesis – the biological process by which an organism develops its shape – has long been a central question in developmental biology. Classical paradigms of morphogenesis emphasize genetic programs, signaling gradients, and mechanical forces that orchestrate cell differentiation and tissue patterning. These traditional views, however, face a fundamental puzzle: DNA alone does not directly encode anatomy ( The bioelectric code: An ancient computational medium for dynamic control of growth and form - PMC ). For example, the genome specifies proteins, but it does not contain a literal blueprint of a hand or a heart. Thus, explaining how complex, large-scale anatomical structures emerge requires understanding multi-level encoding and control systems beyond the gene sequence ( The bioelectric code: An ancient computational medium for dynamic control of growth and form - PMC ) ( The bioelectric code: An ancient computational medium for dynamic control of growth and form - PMC ). While molecular biology has illuminated gene regulatory networks and morphogen pathways underlying development, many organisms exhibit pattern homeostasis – the ability to regulate and restore correct anatomical structure even under novel conditions ( The bioelectric code: An ancient computational medium for dynamic control of growth and form - PMC ). This suggests the existence of additional layers of biological information and control (a “morphogenetic code”), which guide cells and tissues toward specific target forms ( The bioelectric code: An ancient computational medium for dynamic control of growth and form - PMC ).
Recent breakthroughs by Michael Levin and colleagues have introduced a paradigm shift, integrating bioelectric signaling and the concept of cellular cognition into our understanding of morphogenesis. Levin’s research reveals that all cells (not just neurons) use endogenous bioelectric signals – voltage gradients and ion flows – as a computational medium to coordinate growth and form (Frequently Asked Questions - Latest Version). These electrical cues form a “bioelectric code” that can store pattern information and even override genetic default outcomes (Scientists Grow Flatworm With Two Heads Instead Of Tail | IFLScience). Remarkably, cellular collectives exhibit goal-directed behaviors, acting as if they have a built-in memory of the correct anatomical structure and can pursue that target morphology despite perturbations ( The bioelectric code: An ancient computational medium for dynamic control of growth and form - PMC ) ( The bioelectric code: An ancient computational medium for dynamic control of growth and form - PMC ). This thesis integrates classical developmental biology with these radical new insights. We will review the traditional mechanisms of morphogenesis (genetic, epigenetic, biochemical, and biomechanical), then explore Levin’s contributions – the bioelectrical code, pattern memory, and cellular agency in morphogenesis. We compare bioelectric versus genetic determinism, examine case studies (limb regeneration, cancer as a loss of pattern coordination, and synthetic lifeforms like Xenobots), and discuss implications for the origin of form, biological intelligence, and even consciousness. Finally, we analyze open questions and future prospects, including the visionary concept of “anatomical compilers” for top-down control of tissue architecture.
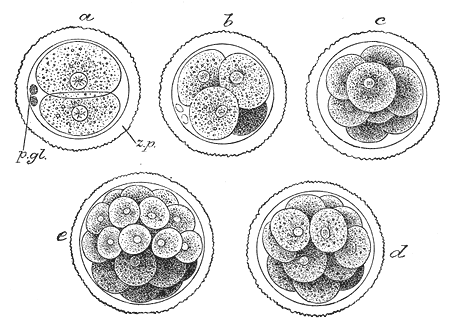
Classical Morphogenetic Mechanisms
Genetic Regulation and the Epigenetic Landscape:
Developmental programs are orchestrated by a genomic “toolkit” of regulators that control cell fates and pattern formation (Morphogenesis - Wikipedia). Master transcription factors and signaling molecules encoded by developmental genes (e.g. Hox genes, Pax genes) activate cascades that define body axes and organ identities (Morphogenesis - Wikipedia). These genetic circuits operate in a hierarchical, feed-forward manner: early embryonic genes define broad domains (e.g. anterior vs. posterior), which in turn activate more specific genes, progressively refining patterns down to individual cell identities ( The bioelectric code: An ancient computational medium for dynamic control of growth and form - PMC ). Conrad Waddington’s famous “epigenetic landscape” metaphor envisioned this process as a marble rolling down branching valleys, where gene-regulatory networks channel cells into particular differentiated states. Modern epigenetics adds that heritable chromatin modifications (DNA methylation, histone marks) lock in these gene expression patterns, stabilizing cell identity during development ( The bioelectric code: An ancient computational medium for dynamic control of growth and form - PMC ). Thus, as cells specialize, they carry an epigenetic memory of their fate – ensuring, for example, a liver cell’s daughter cells remain liver cells. This interplay of genetics and epigenetics establishes the initial conditions for morphogenesis by specifying what cell types form where.
Morphogen Gradients and Signaling Pathways:
A hallmark of classical developmental biology is the concept of morphogens – diffusible molecules that form concentration gradients to pattern tissues (Morphogenesis - Wikipedia). Pioneering work by Lewis Wolpert introduced the “French flag” model, illustrating how a gradient of a factor could subdivide a field of cells into distinct zones (e.g. high morphogen = blue stripe, medium = white, low = red). Empirical examples abound: in the early fruit fly embryo, a Bicoid protein gradient defines the head-tail polarity, while gradients of Wnt, Hedgehog, BMP, and other signaling proteins regionalize tissues in many animals (Morphogenesis - Wikipedia) (Morphogenesis - Wikipedia). Cells interpret morphogen levels via receptors, activating gene expression programs appropriate to their position. Such signaling pathways (Wnt/β-catenin, FGF, Notch, TGF-β/BMP, Hedgehog, etc.) are highly conserved and form interconnected networks that refine the pattern. Morphogen gradients provide a positional information system, telling cells “where” they are so they can differentiate into the correct structure. Importantly, these chemical signals often act in combinatorial fashion – cells integrate multiple gradient inputs to determine fate – and their effects are time-sensitive (exposure duration matters). Together with gene regulatory circuits, morphogen gradients explain how relatively simple initial anisotropies in the egg can amplify into complex spatial patterns across the embryo.
Mechanical Forces and Tissue Dynamics:
Physical forces and biomechanical properties of cells are now recognized as crucial morphogenetic factors. Morphogenesis is inherently a 3D mechanical process involving cell shape changes, movements, and growth under physical constraints (Morphogenesis - Wikipedia). As cells proliferate and migrate, they generate forces that drive tissue bending, folding, extension, and segregation. Classic examples include neurulation (where an epithelial sheet buckles to form the neural tube) and gastrulation (cell layers undergo dramatic movements to form the germ layers). These processes are guided by cytoskeletal contractions, cell adhesion modulation, and differential cell stiffness. Mechanical feedback also influences fate: mechanotransduction pathways (e.g. via YAP/TAZ, integrins) allow cells to sense tissue rigidity or stretch and alter gene expression accordingly (Emerging Role of Mechanical Forces in Cell Fate Acquisition). For instance, stem cells on stiff vs. soft matrices can commit to different lineages. Additionally, tissue-level structures like the extracellular matrix (ECM) provide mechanical cues and scaffolding for morphogenesis. The concept of branching morphogenesis in organs like lungs or kidneys exemplifies how both biochemical signals and mechanical tension shape the complex arborization of tubes (Morphogenesis - Wikipedia). In summary, genetic and biochemical instructions are executed in a physical medium – the embryo – where force, motion, and geometry play instructive roles alongside gene expression. Morphogenesis emerges from this interplay of genetic patterning (“what to build”) and mechanical sculpting (“how to build it”).
Classical Field Models and Feedback:
Earlier theories treated developing tissues as coordinated fields governed by self-organizing principles. Alan Turing’s seminal 1952 paper proposed that interacting chemical substances diffusing and reacting could spontaneously form spatial patterns (the reaction-diffusion model), foreshadowing later discoveries of real morphogen systems (Morphogenesis - Wikipedia). Likewise, the concept of morphogenetic fields (dating back to Hans Driesch and Alexander Gurwitsch, and later popularized by Gurwitsch’s “embryonic fields” and Weiss’s work) suggested that cells respond to global field properties, not just local cues. While these ideas were once considered mystical, modern systems biology incorporates them via feedback loops and emergent dynamics. For example, limb field cells in an embryo collectively decide where a limb will bud, and if part of the field is removed, remaining cells can regulate to still form one complete limb – demonstrating regulative development. Such regulative capacity indicates that cells communicate to fill in missing information and reach a stable pattern. Still, classical models largely attribute this to known biochemical and mechanical feedback. In standard textbooks, morphogenesis is described as the integration of gene regulation, signaling gradients, cell-cell communication, and biomechanics into a progressively unfolding program. By the end of embryogenesis, the organism’s body plan is established through this multi-factorial coordination.
Despite these successes, classical approaches struggle with phenomena like regeneration (how adult tissues reform lost parts) and robust error-correction (how normal anatomy results even in perturbed conditions). These hint that an additional, system-level control is at play. Enter the bioelectric paradigm – an older thread in biology that is now being unraveled with modern techniques, offering new answers to how cells know what to build.
The Bioelectric Code and Cellular Cognition in Morphogenesis
Endogenous Bioelectric Signaling:
Every cell in the body maintains a voltage difference across its membrane (the membrane potential Vmem), and cells can pass current and ions to neighbors through gap junction channels. Developmental bioelectricity refers to the use of these voltage gradients, ion flows, and electric fields as signals that orchestrate pattern formation (Frequently Asked Questions - Latest Version). Remarkably, it has been known for over a century that even non-excitable cells (not just nerves or muscles) exhibit steady bioelectric activity (Frequently Asked Questions - Latest Version). Modern research shows this activity is not just a byproduct of cell physiology, but instructive for cell behaviors during morphogenesis (Frequently Asked Questions - Latest Version). In Levin’s words, “bioelectricity is the computational medium with which cellular collectives make decisions about growth and form.” (Frequently Asked Questions - Latest Version) Like biochemical or genetic information, bioelectric signals form spatiotemporal patterns – for instance, a cluster of cells might be polarized (negative inside) while neighboring tissue is depolarized, creating an electric field pattern. These patterns can act as prepatterns guiding where genes turn on or where growth occurs. Crucially, bioelectric cues often function upstream of gene expression: a change in voltage can trigger downstream transcriptional programs (via voltage-sensitive proteins, calcium signals, etc.), meaning that manipulating bioelectric states can cause large-scale changes in anatomy without altering genes. Bioelectric signaling thus adds a layer of control above the genetic hardware – analogous to a software layer that can write or rewrite the outcome of the genomic program (Frequently Asked Questions - Latest Version) (Scientists Grow Flatworm With Two Heads Instead Of Tail | IFLScience).
Levin has championed the idea of a “bioelectric code”: a set of stable electrical patterns in cell networks that encode anatomical information (much as neural electrical networks encode memories) (Frequently Asked Questions - Latest Version). During embryogenesis and regeneration, cells appear to use bioelectric signals to communicate their position and to coordinate who should grow what where. For example, prior to facial development in frog embryos, an “electric face” pattern of membrane voltage arises, foreshadowing the locations of eyes, nose, and mouth – perturbing this voltage map can cause craniofacial malformations ( The bioelectric code: An ancient computational medium for dynamic control of growth and form - PMC ). Such evidence suggests that bioelectric patterns function as instructive prepatterns for morphology. In planar cell networks, different voltage domains can be thought of like a “blueprint” – except it’s a physiological blueprint that can be dynamically updated. Importantly, whereas DNA is a linear code (1D sequence) and biochemical gradients are largely diffusive (3D concentrations), bioelectric patterns have a truly 4D character (spread over 3D tissues and time) and can be rapidly changed or propagated, more akin to a computational medium. This makes them suitable for implementing feedback, memory, and real-time adjustments during growth ( The bioelectric code: An ancient computational medium for dynamic control of growth and form - PMC ) ( The bioelectric code: An ancient computational medium for dynamic control of growth and form - PMC ).
Bioelectric Circuits and Pattern Memory:
Multicellular tissues can maintain stable bioelectric states over time, effectively storing information about the target anatomy (Frequently Asked Questions - Latest Version). Just as neural networks store memories in electrical activity patterns, cell collectives outside the brain also exhibit long-term stable electrical patterns that can serve as pattern memories (Frequently Asked Questions - Latest Version) (Frequently Asked Questions - Latest Version). Levin’s experiments with planarian flatworms dramatically demonstrated this. Planaria normally regenerate a head and tail in correct positions when cut. By experimentally “rewiring” the worm’s bioelectric circuit (e.g. using drugs or genetic tools to alter ion channel activity), one can permanently change the body pattern that the worm’s cells rebuild. In one study, brief exposure to a gap junction blocker caused planaria to regenerate with two heads (one at each end) instead of a head-tail configuration ( The bioelectric code: An ancient computational medium for dynamic control of growth and form - PMC ) (Scientists Grow Flatworm With Two Heads Instead Of Tail | IFLScience). Remarkably, these two-headed worms remember this pattern: when cut again, they regenerate two heads again, even without further treatment, indicating that a new pattern memory has been imprinted in the tissue ( The bioelectric code: An ancient computational medium for dynamic control of growth and form - PMC ). The altered bioelectric state (depolarized at both ends) effectively re-specifies the target morphology that cells aim to construct ( The bioelectric code: An ancient computational medium for dynamic control of growth and form - PMC ). Only by resetting the electrical state (for instance, with treatments that re-normalize ion flows) can the worms be restored to one-headed regenerations ( The bioelectric code: An ancient computational medium for dynamic control of growth and form - PMC ). In another experiment, Levin’s team managed to induce flatworms of one species to regenerate the head shape of a different species by modulating bioelectric signals, without any genomic changes ( The bioelectric code: An ancient computational medium for dynamic control of growth and form - PMC ). These results are profound: they show that the same genetic hardware can build different anatomical outcomes depending on the bioelectric patterning signals. In essence, the bioelectric code is an additional set of instructions on top of the genetic code, and it can override the default genetic body plan under certain conditions (Scientists Grow Flatworm With Two Heads Instead Of Tail | IFLScience).
Bioelectric pattern memory blurs the line between developmental biology and neural memory. It suggests that tissues have basal cognitive properties: they can store a representation of a shape and actively work to achieve or maintain it. For example, when a salamander’s limb is amputated, the cells in the stump do not simply follow a hardcoded linear script; rather, they seem to assess the deviation from the normal limb pattern and execute actions to restore it – like a thermostat with a setpoint (the setpoint being the correct limb shape). This kind of process implies that the regenerative cells have access to an internal model or memory of the proper anatomy (a limb blueprint), and will keep building until that blueprint is achieved. Levin has described this as the tissue having a target morphology that it tries to reach, using whatever cellular mechanisms are available ( The bioelectric code: An ancient computational medium for dynamic control of growth and form - PMC ). The bioelectric code is a prime candidate for where that target morphology is stored and continually referenced during pattern correction.
Cellular Decision-Making and Goal-Directed Growth:
Levin’s framework goes further to propose that cell groups exhibit a form of primitive intelligence or cognitive behavior during morphogenesis (Bioelectric networks: the cognitive glue enabling evolutionary scaling from physiology to mind | Animal Cognition ) (Frequently Asked Questions - Latest Version). Of course, individual cells do not have neurons or brains, but they do have computational abilities – they sense their environment (chemicals, neighbors, electric fields, etc.), make internal decisions (through signaling networks), and act (changing shape, moving, dividing). When many cells interact, especially via electrical synapses (gap junctions) and shared voltage fields, the collective can perform complex information-processing. Levin calls this the collective intelligence of cellular swarms, and suggests it is truly cognitive in that it handles goals and memories (Frequently Asked Questions - Latest Version) (Bioelectric networks: the cognitive glue enabling evolutionary scaling from physiology to mind | Animal Cognition ). The “goal” in development is the correct anatomical outcome, and the system is remarkably robust in achieving it. For instance, if part of an embryo is removed or rotated, often the remaining cells regulate to still form a complete organism (as in classic experiments grafting two halves of newt embryos together to get a single embryo) ( The bioelectric code: An ancient computational medium for dynamic control of growth and form - PMC ). Such regulation implies a top-down control – cells are coordinating towards a higher-level outcome (the body plan) rather than just following local rules blindly. This coordination can be seen as an example of emergent, distributed problem-solving. The cells are cooperating to “solve” the task of building an organ, similar to how ants in a colony solve building a nest without a central controller.
Bioelectric signals are uniquely suited to this kind of collective decision-making. Electrical propagation is fast and can integrate over large distances in the tissue, meaning cells far apart can influence each other in real time. Levin refers to bioelectric networks as the “proto-cognitive medium” that evolution discovered long before brains (Frequently Asked Questions - Latest Version). In effect, evolution had a working system for storing information and orchestrating activity across cell groups using ion channels and voltage changes, hundreds of millions of years before specialized neurons appeared (Frequently Asked Questions - Latest Version). Brains are essentially a later specialization of this ancient electrical communication system. This deep continuity suggests that the difference between developmental morphogenesis and conventional animal behavior is one of degree, not kind (Bioelectric networks: the cognitive glue enabling evolutionary scaling from physiology to mind | Animal Cognition ). Indeed, Levin notes a “deep symmetry between the intelligence of developmental morphogenesis and that of classical behavior” (Bioelectric networks: the cognitive glue enabling evolutionary scaling from physiology to mind | Animal Cognition ) – both involve ensembles of agents (cells or neurons) communicating and coordinating to achieve functional outcomes (anatomy or behavior). This perspective recasts morphogenesis as not just a mechanistic unfolding of a genetic program, but as a process of constraint satisfaction and error minimization carried out by cells with limited goals and information. The cells themselves can be seen as having “agendas” – e.g. a cell might “try” to close a wound or to contribute to a tube’s curvature – and these local goals sum up to the global anatomy.
In summary, Levin’s work elevates the importance of bioelectric signaling as a master regulator of form and introduces the notion of cells as intelligent agents of morphogenesis. Pattern formation is thus both a biophysical process (involving voltage and ion flows) and a computational one (involving information processing and feedback). The next sections will delve into specific comparisons and cases that highlight how this bioelectric view contrasts with genetic determinism and how it explains complex phenomena like regeneration, cancer, and creation of synthetic life forms.
Bioelectricity versus Genetic Determinism
A key paradigm shift in understanding morphogenesis is moving from a gene-centric determinism to a multi-factorial, information-centric view. In the classical view, the genome is often treated as the “blueprint” of the organism, implying that all necessary information for anatomy is in DNA. In contrast, the bioelectric view asserts that the genome is just one component – providing the parts list (proteins) and lower-level rules – while the actual blueprint (or construction plan) is an emergent property of cell interactions, of which bioelectric communication is crucial ( The bioelectric code: An ancient computational medium for dynamic control of growth and form - PMC ) (Frequently Asked Questions - Latest Version).
It is not genes vs. electricity, but rather genes and electricity (and mechanics, etc.) in a hierarchical relationship. Genes code for ion channels, receptors, adhesion molecules, etc., which make bioelectric and other signals possible. But once those components are in place, the collective signals can exert higher-level control back onto gene expression. For example, activating a certain ion channel gene (genetic change) can set a cell’s voltage high or low; conversely, forcing a cell’s voltage high or low (electrical change) can activate or repress many genes (via voltage-sensitive response elements). Thus, a feedback loop connects genotype and bioelectric state. Genetic determinism tends to assume a mostly feed-forward flow of information (DNA → RNA → protein → structure), whereas the integrated view recognizes feedback (structure or physiological state → gene expression) and distributed control. As Levin writes, “pattern control involves a code” that is not reducible to DNA sequence alone ( The bioelectric code: An ancient computational medium for dynamic control of growth and form - PMC ). DNA encodes hardware (proteins) for single cells, but the software of anatomy – the instructions for assembling those parts into organs – is at least partly in the electrical domain of cell networks (Frequently Asked Questions - Latest Version).
Indeed, we currently cannot predict an organism’s detailed anatomy from its genome alone (Frequently Asked Questions - Latest Version). A thought experiment from Levin: if you mix cells from a frog and an axolotl (salamander) to create a chimera (sometimes nicknamed a “frogolotl”), could you predict whether it will develop legs, or what the hybrid will look like? The answer is no – having both genomes doesn’t directly tell us the anatomical outcome (Frequently Asked Questions - Latest Version). This underscores that genomic information is necessary but not sufficient to determine form. By contrast, if one could read the bioelectric state map of an early embryo, one might have a much better prediction of the morphology that will result (since that map encodes the plan). In essence, genetic determinism is incomplete: it overlooks higher-level regulators like physiological circuits.
Bioelectricity also offers an explanation for why multiple genetic or biochemical pathways can compensate for each other to achieve the same morphology. In developmental biology, researchers often find that knocking out a single gene does not always produce a dramatic anatomical defect – often because of redundancy or alternative pathways. Levin and others note an “implementation independence” principle in development: diverse molecular mechanisms can lead to the same anatomical end-state (Top-down models in biology: explanation and control of complex living systems above the molecular level | Journal of The Royal Society Interface). What this suggests is a high-level setpoint (the target anatomy) that the system will reach via any of several lower-level routes. Bioelectric circuits could be a coordinator of this process, ensuring that no matter which molecular pathway is used, the collective will stop when the correct structure is achieved. This is analogous to how an engineer cares about the final shape of a robot, not exactly which screw got tightened first – multiple assembly sequences can yield the same product. In biology, cells with different gene expression profiles can still coordinate to form the same organ if the overarching pattern signals are in place (Top-down models in biology: explanation and control of complex living systems above the molecular level | Journal of The Royal Society Interface). This “many roads to Rome” aspect is hard to reconcile with strict genetic determinism but fits a model where genetic, biochemical, and bioelectric layers jointly implement a robust architecture with goal-seeking behavior (Top-down models in biology: explanation and control of complex living systems above the molecular level | Journal of The Royal Society Interface) (Top-down models in biology: explanation and control of complex living systems above the molecular level | Journal of The Royal Society Interface).
Another contrast is in how we view errors and corrections. Genetic determinism struggles to explain how an organism corrects deviations (since a strict program would not have flexibility for large perturbations), whereas a bioelectric view naturally includes feedback that can correct or adjust patterns dynamically (because cells continuously communicate and update their state). Consider the phenomenon of monozygotic twins: one embryo splits into two, and each half regenerates the missing parts to form two complete individuals. The original genome was for one individual, yet when physically halved, each half scales and repatterns to generate a full body ( The bioelectric code: An ancient computational medium for dynamic control of growth and form - PMC ). This indicates that after the split, cell groups knew that they only had half the required body and coordinated a fix – something that wasn’t explicitly “in the DNA” per se. Pattern homeostasis via bioelectric and biochemical feedback loops can account for such regulative development ( The bioelectric code: An ancient computational medium for dynamic control of growth and form - PMC ).
To be sure, genes are absolutely crucial: they define the components of the bioelectric circuits (e.g., what ion channels are present) and thus set the possibilities for the bioelectric code. But Levin’s insights add that editing physiological state can sometimes trump genetics in outcome. A striking statement from his lab: “Bioelectric properties can permanently override the default body shape called for by a genome” (Scientists Grow Flatworm With Two Heads Instead Of Tail | IFLScience). In the two-headed worm, the genome still encoded a one-headed worm, yet the bioelectric override produced a stable two-headed phenotype (Scientists Grow Flatworm With Two Heads Instead Of Tail | IFLScience). This does not violate genetics, but it shows the genome’s effects are interpreted by cell networks, and that interpretation can change. In summary, the relationship between genes and bioelectricity is akin to hardware and software: one provides the devices, the other the programmability. Thinking in this way moves us beyond a one-way chain of causation to a cybernetic view of development, where feedback, memories, and high-level control are integral.
Case Studies Integrating Bioelectric and Classical Perspectives
Regeneration of Limbs and Other Structures
One of the most vivid demonstrations of morphogenetic control is the regeneration of lost body parts in certain animals. Classical biology has documented how salamanders, for example, can regrow amputated limbs, tails, jaws, even parts of the heart and brain ( The bioelectric code: An ancient computational medium for dynamic control of growth and form - PMC ). Planarian flatworms can regenerate an entire organism from just a tiny fragment – a dramatic case of morphogenetic resilience ( The bioelectric code: An ancient computational medium for dynamic control of growth and form - PMC ). Understanding regeneration pushes the limits of our knowledge: how do adult cells reconstitute the precise pattern of a complex appendage?
Traditionally, regeneration has been studied in terms of dedifferentiation (mature cells near the wound revert to a stem-like state), formation of a proliferative blastema, and redeployment of embryonic signaling pathways (like BMP, Wnt, FGF, etc.) in the stump. These factors indeed play critical roles. But an often overlooked aspect was the bioelectric environment of the wound and regenerating tissue. It has been observed since the 1800s that injury currents and ion fluxes occur at wound sites. In regenerative species, the wound quickly establishes an ion current and voltage gradient that is required for regeneration. Levin’s work showed causative roles for specific ion transporters: e.g., in Xenopus (African clawed frog) tadpoles, a proton pump (V-ATPase) creates a localized membrane voltage change that is necessary and sufficient to trigger tail regeneration ( The bioelectric code: An ancient computational medium for dynamic control of growth and form - PMC ). By hyperpolarizing cells on an Xenopus tail stump (using a light-activated ion pump), they could induce regeneration even in older froglets that normally have lost regenerative ability ( The bioelectric code: An ancient computational medium for dynamic control of growth and form - PMC ). This indicates that bioelectric triggers can re-awaken latent regenerative programs.
Levin’s lab also demonstrated that changing the bioelectric state can produce new growth where it normally wouldn’t occur. In one experiment, misexpression of certain ion channels in Xenopus embryos caused the formation of ectopic eyes – eyes developing in the gut, tail, or back of the tadpole. Amazingly, these eyes had reasonably correct structure and even connected to the nervous system. The cue that initiated them was a bioelectric pattern mimicking the one in the face that induces eye formation (Frequently Asked Questions - Latest Version). This shows the instructive power of bioelectric signals for complex organogenesis.
Perhaps the most significant recent application was in inducing limb regeneration in a non-regenerative animal. Adult frogs (post-metamorphic Xenopus) normally heal limb amputations with a simple cartilaginous spike, not a full limb. In 2022, Nirosha Murugan, Levin, and collaborators reported a breakthrough: by using a wearable bioreactor “BioDome” loaded with a cocktail of drugs that modulate early signaling (including ion channel modulators), they triggered 18 months of regrowth, resulting in an appendage with bones, nerves, muscles, and some functional restoration ( Acute multidrug delivery via a wearable bioreactor facilitates long-term limb regeneration and functional recovery in adult Xenopus laevis - PMC ) ( Acute multidrug delivery via a wearable bioreactor facilitates long-term limb regeneration and functional recovery in adult Xenopus laevis - PMC ). Notably, the treatment was only 24 hours long, but it “kick-started” a self-limited developmental program that unfolded over months to gradually build the limb structure ( Acute multidrug delivery via a wearable bioreactor facilitates long-term limb regeneration and functional recovery in adult Xenopus laevis - PMC ) ( Acute multidrug delivery via a wearable bioreactor facilitates long-term limb regeneration and functional recovery in adult Xenopus laevis - PMC ). The induced limb was not perfect, but it was far more complex than a spike – including several toes and moveable joints. Analysis showed activation of developmental pathways (Wnt, TGF-β, hedgehog, Notch) in the early bud, indicating that a cascade akin to embryonic limb development had been launched ( Acute multidrug delivery via a wearable bioreactor facilitates long-term limb regeneration and functional recovery in adult Xenopus laevis - PMC ). This case exemplifies using a top-down induction: rather than micromanaging every growth step, the intervention provided an initial set of information-rich triggers and a permissive environment (the BioDome kept the wound moist and drug-enriched) to let cells do the rest ( Acute multidrug delivery via a wearable bioreactor facilitates long-term limb regeneration and functional recovery in adult Xenopus laevis - PMC ). In essence, it gave cells an instructive nudge (“start building a limb”) and then the cellular collective intelligence carried out the goal over a long term.
Regeneration thus highlights the pattern memory concept: the remaining tissue after amputation somehow “knows” the correct pattern to rebuild (a limb of proper size and shape). If that knowledge can be unlocked (as by electrical cues) even non-regenerative species might be coaxed to regenerate. Conversely, if pattern memory is disrupted, regeneration fails or goes awry. For example, if the electrical signal at a wound is scrambled, planaria might regenerate with the wrong morphology or not at all (Scientists Grow Flatworm With Two Heads Instead Of Tail | IFLScience). Indeed, Levin’s team concluded that “bioelectrical patterns determine what the flatworms will become after they’ve regenerated – rather than [just] stem cells” (Scientists Grow Flatworm With Two Heads Instead Of Tail | IFLScience). The stem cells provide raw material, but it’s the electrical pattern that instructs the shape of the new growth. From a classical standpoint, we add that the bioelectric cues ultimately converge on classical pathways (turning genes on/off, etc.), but they do so in a coordinated, large-scale fashion that gene networks alone find hard to achieve.
In summary, limb and tissue regeneration demonstrate the power of integrating bioelectric and classical views: local biochemical signals and stem cell responses operate within a global bioelectric coordinate system that tells the cells what pattern to rebuild. Studying regeneration with this lens is leading to new “electroceutical” or morphoceutical strategies – using ion channel drugs or electrical stimulation to induce desired growth. This could revolutionize regenerative medicine by moving beyond gene therapy to physiological therapy that leverages the body’s own patterning intelligence.
Cancer as a Disorder of Cellular Communication (Cognitive Dissociation)
Cancer is traditionally seen as a genetic disease: mutations accumulate in a cell lineage, causing cells to proliferate uncontrollably and invade tissues. While genetic mutations are clearly involved, Levin’s perspective adds a new dimension: cancer may be a developmental disorder – a loss of the normal bioelectric and communication controls that keep cells working toward the organism’s morphological goals. In other words, cancer cells are healthy cells that have become disconnected from the multicellular plan and have reverted to a more single-cell agenda (growth and migration, ignoring the body’s patterning cues).
There is evidence that disrupting bioelectric signals can induce a cancer-like state even without introducing oncogenes. For instance, research showed that altering the resting potential of cells (depolarizing them inappropriately) can trigger cells to start forming tumors in Xenopus tadpoles ( The bioelectric code: An ancient computational medium for dynamic control of growth and form - PMC ). One study by Chernet and Levin found that introducing specific ion channel misexpression led to metastatic behavior in otherwise normal cells ( The bioelectric code: An ancient computational medium for dynamic control of growth and form - PMC ). These findings align with the idea that cells interpret a drastically aberrant electrical environment as a cue to break away from the tissue’s cooperative program – essentially, a cellular “confusion” that leads to cancer. Normally, cells in an organ are electrically coupled and share signals that reassure each cell that “all is well” and they are in the correct location. If those signals are lost (say, by a mutation that closes gap junctions or changes ion channel activity), a cell might behave as if it’s in the wrong context – triggering developmental programs that are inappropriate (like proliferation or migration, as if it were healing a wound or building a structure that doesn’t actually belong).
Levin describes cancer as “cells defecting from the body’s patterning cues” ( The bioelectric code: An ancient computational medium for dynamic control of growth and form - PMC ). In a healthy state, each cell’s activity is constrained by the collective goal (maintaining tissue architecture). When that restraint is lifted, cells treat the body as just environment to exploit, and we see tumor growth. The bioelectric model of cancer predicts that re-normalizing the bioelectric environment could suppress tumorigenesis. Indeed, experiments have shown that forced hyperpolarization of tumors (using ion channel drugs that make cancer cells more electrically negative inside) can cause them to stop proliferating or even undergo apoptosis, essentially re-integrating them into the normal program (Bioelectric networks: the cognitive glue enabling evolutionary scaling from physiology to mind | Animal Cognition ). For example, in one Xenopus tumor model, misexpression of a certain channel made cells cancerous, but co-expression of a hyperpolarizing channel (restoring a normal voltage) significantly reduced abnormal growth ( The bioelectric code: An ancient computational medium for dynamic control of growth and form - PMC ). Such results hint at future non-genetic cancer therapies: electroceutical approaches that “persuade” cancer cells back into compliance. This is conceptually akin to treating cancer as a biophysical communication problem rather than solely a DNA mutation problem.
Another striking aspect is developmental bioelectric signals are involved in processes like left-right patterning and organ shape; cancer could be seen as these processes gone wrong. Levin and colleagues even speculated that some cancers might be an atavism – cells trying to form a “primitive” or inappropriate structure (like a tumor that expresses embryonic patterns). By restoring correct bioelectric cues, you remind the cells of their actual role in the current body plan. As one paper title by Levin’s group put it: “oncogene-induced tumorigenesis and metastasis: the bioelectric basis of cancer in vivo.” ( The bioelectric code: An ancient computational medium for dynamic control of growth and form - PMC ) Recognizing cancer as a developmental (patterning) disease opens new research questions: Is there a bioelectric signature that universally distinguishes tumor cells from normal? Can we detect early tumors via voltage imaging? Can we normalize a neoplasm by coupling it electrically to healthy tissue? Early studies are promising – for instance, injecting dye that reports voltage showed distinct electrical properties of tumor sites in a frog model, and intervention on those properties halted tumor progression (Bioelectric networks: the cognitive glue enabling evolutionary scaling from physiology to mind | Animal Cognition ).
In summary, integrating Levin’s view, we can say cancer is a loss of morphogenetic information sharing – essentially a break in the collective intelligence of cells. Normal tissues suppress individual cell ambitions in favor of the anatomical plan; cancer cells “go rogue” by escaping that suppressive, instructive field. They are less like a different species of cell (genetically they’re mostly the same as normal) and more like normal cells in the wrong software state. This radically different framing sees tumors as a reversible state (in principle), which could be corrected by reconnecting the cells into the body’s bioelectric network (Bioelectric networks: the cognitive glue enabling evolutionary scaling from physiology to mind | Animal Cognition ). It also connects cancer to the theme of cellular cognition: just as a failing in brain networks can produce aberrant behaviors, a failing in somatic cell networks produces aberrant growths.
Synthetic Lifeforms: Xenobots as Morphological Novelty
A dramatic illustration of cells’ developmental plasticity and intelligence came with the creation of Xenobots in 2020. These are synthetic living “robots” assembled entirely from frog (Xenopus) embryonic cells, designed by an evolutionary algorithm to perform simple tasks (Scientists Assemble Frog Stem Cells Into First 'Living Machines'). Scientists took dissociated frog stem cells (which normally would build a tadpole if arranged in an embryo) and recombined them in novel configurations guided by a computer simulation. The result was tiny (~millimeter-sized) organisms unlike any seen in nature: neither frog nor traditional machine, but living blobs that could move, work together, and even repair themselves (Scientists Assemble Frog Stem Cells Into First 'Living Machines'). For example, one xenobot design consisted of an arrangement of skin epithelial cells (providing a passive body structure) and heart muscle cells (providing spontaneous contractile force) (Scientists Assemble Frog Stem Cells Into First 'Living Machines'). The muscle contractions allowed the organism to “swim” around in a Petri dish. These xenobots were able to “zoom around”, push small particles to aggregate them, and if cut in half, they healed back together (Scientists Assemble Frog Stem Cells Into First 'Living Machines'). Notably, they had no neurons or sensory organs, yet their behavior appeared coordinated – a testament to the inherent abilities of cells. Levin calls them “a new form of life… somewhere in between an organism and a machine” (Scientists Assemble Frog Stem Cells Into First 'Living Machines').
From a morphogenesis standpoint, Xenobots are fascinating because they show how cells can be reconfigured to produce radically different anatomies and functions without genetic changes. The cells used to make xenobots all have the normal frog genome, yet when freed from the frog embryo’s usual context, they self-organize into a completely different morphology (Scientists Assemble Frog Stem Cells Into First 'Living Machines'). Skin cells, which in a frog embryo would form a static outer layer, instead formed a spheroid that worked as a coherent locomotive unit. The heart cells, instead of pumping blood, acted as little engines. This demonstrates a key point: cells are not hard-wired to build only the organs evolution assigned them; rather, they respond to local signals and will cooperate to create an ensemble that makes sense under the circumstances. In the case of xenobots, the circumstances (a ball of cells with no nutrient supply) led them to form a ciliated surface for locomotion and a behavior of pushing pellets (perhaps analogous to clearing debris or attempting to aggregate food, although the exact reason for that behavior is still studied). It is an example of cells’ programmability by configuration.
Xenobots also surprised the world when a follow-up study showed they could even exhibit a form of kinematic self-replication. When many xenobots were placed with loose frog cells, the xenobots ended up corralling those cells into piles which then matured into new xenobots – essentially reproducing in a way not seen in known organisms (Kinematic self-replication in reconfigurable organisms - PNAS). A “swarm of frog-cell parents” pushing cells into a heap that becomes “children” is a striking reminder that life’s rules are broader than traditional biology when cells are given new contexts (Kinematic self-replication in reconfigurable organisms - PNAS). This is relevant to morphogenesis because it suggests that the capacity for certain behaviors (like collective motility or aggregation) lay dormant in those cells, only to be revealed under appropriate configurations. Evolution in nature never had frog cells experiencing that context, so this replication method was never observed – yet it was always possible, as the xenobots showed.
What do xenobots teach us about the origin of form? They show that form is remarkably plastic. The same genotype can yield different anatomies; the outcome depends on cellular interactions and initial conditions. Normally, the frog genome + frog developmental environment gives you a tadpole. But frog genome + human-designed assembly gave us a xenobot. Notably, the design of xenobots was done with the help of an AI that tried various shapes in simulation to achieve a goal (e.g. locomotion). This hints at the future: using computational tools to explore the space of possible multicellular forms (the “morphospace”). Xenobots sit at the intersection of biology and engineering – they are living testbeds for theories of morphogenesis. If one can systematically vary their designs and observe outcomes, one can learn how certain configurations lead to self-organization of particular structures or behaviors, essentially probing the rules of cellular cooperation.
From Levin’s perspective, xenobots demonstrate cells behaving as an agential collective: deprived of their usual developmental instructions, they still spontaneously do something functional (move, aggregate), rather than just forming a formless blob. This implies that cells have built-in defaults and rules of engagement that produce ordered structure even outside normal developmental channels – supporting the idea of cells having agendas (like “stick together and move”).
In summary, xenobots underscore the theme that anatomy is not hardcoded in DNA – DNA provides subroutines, but the actual form emerges from cell interactions which can be directed in unconventional ways. They also serve as a prototype for synthetic morphology: perhaps in the future, we might design bespoke organisms or tissues to perform tasks (biomedical or environmental). Lessons from xenobots may inform the development of the aforementioned “anatomical compiler” (discussed later) by showing how far we can push cells to create new forms when given novel instructions.
(Scientists Assemble Frog Stem Cells Into First 'Living Machines') Figure: A “xenobot” assembled from frog skin (green) and heart muscle cells (red). Although genetically identical to a frog’s cells, this living machine has a novel form and behavior, demonstrating the plasticity of cellular collectives (Scientists Assemble Frog Stem Cells Into First 'Living Machines') (Scientists Assemble Frog Stem Cells Into First 'Living Machines').
Theoretical Implications for the Origin of Form and Biological Intelligence
The synthesis of traditional morphogenesis with bioelectric and cognitive concepts has deep implications for fundamental questions:
The Origin of Biological Form:
How do shapes and body plans arise and evolve? The classical gene-centric view would say mutation and selection act on genes that change developmental processes, leading to new forms. The integrated view adds that evolution doesn’t directly “code” for final anatomy but rather for systems of cell cooperation. The genome specifies a starting configuration and components (cells, signals) and a set of rules (e.g. gene regulatory networks, bioelectric coupling parameters). The final form arises from the execution of these rules, which may involve a degree of self-organization. This means evolution might often change higher-level parameters (like the resting potential of a tissue, or the connectivity of cells) to alter form, in addition to changing protein sequences. It also means some forms are easier to evolve than others – those that are attractors of the developmental system’s dynamics. Viewing morphogenesis as an attractor-seeking process (with target morphology as an attractor state) helps explain robustness and also how radically different forms can evolve by tweaking the system’s initial conditions or feedback laws rather than redesigning from scratch. Levin’s work even suggests that evolution has exploited the modularity afforded by the bioelectric code: because similar bioelectric patterns can be produced by different molecular mechanisms, organisms can evolve new shapes without needing completely new genes – they can reuse existing channels and signals in new configurations (Top-down models in biology: explanation and control of complex living systems above the molecular level | Journal of The Royal Society Interface). This could partly explain phenomena like convergent evolution (different lineages arriving at similar forms via different genes) or the mystery of how rapid leaps in form (macroevolutionary jumps) might be achievable by rewiring cell communication rather than waiting for numerous mutations.
The origin of form is thus reframed: it’s about how collective cell behaviors are coordinated. Genes set up the playground; the game is played via bioelectric, chemical, and mechanical information exchange. It resonates with older ideas of morphogenetic fields – now we can think of fields concretely as electrical and chemical gradients that impose order. It also has echoes in D’Arcy Thompson’s notion that physical forces mold forms – here, we have a richer set of “forces” including electrical potentials and cognitive-like feedback forcing the system toward a shape.
Biological Intelligence and “Primitive” Cognition:
If we accept that cell groups make decisions in morphogenesis, we are essentially attributing a form of intelligence (albeit rudimentary) to living tissues. This idea falls under the umbrella of basal cognition – the study of how even simple organisms or collectives process information and exhibit behaviors analogous to learning, memory, and problem-solving (Bioelectric networks: the cognitive glue enabling evolutionary scaling from physiology to mind | Animal Cognition ). Levin and colleagues argue that all living things partake in some degree of cognition, defined as the ability to sense conditions, evaluate options, and take actions to meet goals (Bioelectric networks: the cognitive glue enabling evolutionary scaling from physiology to mind | Animal Cognition ). In multicellular development, the “goal” is usually the correct body structure (and during regeneration, the goal is to restore the original pattern). They point out that all intelligence is collective intelligence at some level – a human brain’s intellect emerges from billions of neurons, which themselves are cells; likewise an embryo’s morphogenesis emerges from trillions of cells interacting (Bioelectric networks: the cognitive glue enabling evolutionary scaling from physiology to mind | Animal Cognition ). The continuum from simple homeostatic loops (a cell maintaining its internal environment) to complex behaviors (an animal finding food) is seen as a continuous scaling of problem-solving competency (Bioelectric networks: the cognitive glue enabling evolutionary scaling from physiology to mind | Animal Cognition ). In Levin’s words, the “remarkable trick of evolution” was to take the same basic processes that let cells build bodies and reuse them to let organisms behave in the world (Bioelectric networks: the cognitive glue enabling evolutionary scaling from physiology to mind | Animal Cognition ). The navigation of morphospace (the space of possible forms) by cells as they construct an embryo is directly analogous to the navigation of 3D space by an animal seeking goals (Bioelectric networks: the cognitive glue enabling evolutionary scaling from physiology to mind | Animal Cognition ). This is a profound conceptual bridge between developmental biology and neuroscience.
One implication is that studying how cells cooperate to build organs could inform understanding of how neurons cooperate to produce thoughts – and vice versa. Indeed, techniques of neuroscience (like network modeling, information theory, and even interventions like optogenetics) are being applied to non-neural tissues to decode the “body’s internal communication” (Frequently Asked Questions - Latest Version) (Bioelectric networks: the cognitive glue enabling evolutionary scaling from physiology to mind | Animal Cognition ). The hope is that by cracking the bioelectric code, we not only gain control over growth and form, but we also learn general principles of collective intelligence that might apply to swarms, ecosystems, or new AI algorithms. It reframes morphogenesis as a kind of emergent computation performed by cell collectives.
Consciousness (Speculative):
While highly speculative, some have wondered if the continuum of cognition extends to actual experience or consciousness in simpler systems. Levin tends to avoid grand claims about cell consciousness in his scientific work (Forms of life, forms of mind | Dr. Michael Levin | Videos of, and transcripts of Q&A after, a few of my recent talks), focusing on objectively observable competencies. However, he has mused that since we attribute consciousness to brains because of their complex electrical activity, perhaps under certain conditions, other electrical networks (e.g. organ systems) could have rudimentary analogs of it (Forms of life, forms of mind | Dr. Michael Levin | Videos of, and transcripts of Q&A after, a few of my recent talks). This does not mean your liver is “conscious” in a human sense, but it provokes thought: could there be multiple scales of mind within us (as some philosophies of mind, like panpsychism or integrated information theory, might suggest)? The line of reasoning is that if we see continuity from single-celled behaviors up to human behavior, the emergence of subjective awareness might likewise be a graded, continuum phenomenon rather than an on/off switch that only brains have. The cellular perspective might help demystify consciousness by rooting it in the incremental addition of communication and memory layers. That said, this thesis primarily treats consciousness as beyond its scope – we mention it as a horizon question. The more immediate point is that morphogenesis requires a shift in thinking from seeing cells as tiny robots executing a genetic program to seeing them as agents collaborating in a scheme that has hallmarks of primitive purpose.
Revisiting Teleology in Biology:
The language of “goals” and “information” in developmental biology has historically been controversial, as it can smack of teleology (purpose-driven processes). However, modern theoretical biology is revisiting these terms in rigorous ways. Concepts like attractors, setpoints, homeostasis, and error-correction provide a non-mystical framework to talk about goal-directedness (Top-down models in biology: explanation and control of complex living systems above the molecular level | Journal of The Royal Society Interface). For instance, saying “cells aim to rebuild a limb” can be translated into: the limb-forming system has an attractor state in which a full limb exists, and the dynamics will push the system toward that state from various initial states (with a missing limb being a perturbation that triggers dynamics back to the attractor). This is similar to how we understand physiological homeostasis (the body “seeks” 37°C temperature, but really it’s just feedback loops). By adopting such models, we legitimize discussing top-down causation – higher-level order influencing lower-level events – without invoking any mystical vitalism (Top-down models in biology: explanation and control of complex living systems above the molecular level | Journal of The Royal Society Interface) (Top-down models in biology: explanation and control of complex living systems above the molecular level | Journal of The Royal Society Interface). The bioelectric code is a plausible mechanism for top-down control, as it represents a system-wide state (voltage pattern) that can constrain local cell activity (Top-down models in biology: explanation and control of complex living systems above the molecular level | Journal of The Royal Society Interface) (Top-down models in biology: explanation and control of complex living systems above the molecular level | Journal of The Royal Society Interface).
Future Perspectives: Anatomical Compilers and Top-Down Control
One of the most exciting prospects raised by this integrated understanding is the development of anatomical compilers – a concept championed by Levin. The idea of an anatomical compiler is inspired by computer science: just as a software compiler takes a high-level description of a program and translates it into machine code that the hardware can execute, an anatomical compiler would take a high-level description of a desired organ or body structure and figure out the low-level stimuli to apply to cells so that they build that structure (Forms of life, forms of mind | Dr. Michael Levin | Videos of, and transcripts of Q&A after, a few of my recent talks). In Levin’s vision, you could literally “print” new forms by specifying what you want and letting the compiler compute the sequence of bioelectric (and biochemical) instructions to make cells create it (Forms of life, forms of mind | Dr. Michael Levin | Videos of, and transcripts of Q&A after, a few of my recent talks). For example, one might say “build me a six-legged frog with wings” (a whimsical example he gives) and the compiler would output the cocktail of changes to the frog embryo’s bioelectric pattern (and other signals) to achieve that (Forms of life, forms of mind | Dr. Michael Levin | Videos of, and transcripts of Q&A after, a few of my recent talks). While this sounds fantastical, the underpinning is our increasing knowledge of control knobs in morphogenesis. We already have some knobs: we know certain ion channel cocktails that trigger limb regrowth ( Acute multidrug delivery via a wearable bioreactor facilitates long-term limb regeneration and functional recovery in adult Xenopus laevis - PMC ), certain voltage changes that induce an eye or a brain in new locations, certain gene circuits that form extra segments, etc. The compiler approach would use algorithms (possibly AI) to explore combinations of such interventions to reach a target outcome.
Achieving this requires both fundamental knowledge and advanced technology. We need a much fuller map of the bioelectric code: i.e., what pattern corresponds to a limb vs. a tail vs. an eye, etc., in various species. We also need real-time, high-resolution voltage imaging and optogenetic control so that we can read and write patterns as needed. And critically, we need to integrate bioelectric cues with biochemical and mechanical context – the compiler might output multi-modal instructions (e.g., apply this voltage, at this time add FGF, provide a scaffold of this stiffness, etc.). In current reality, researchers are already experimenting with such multi-modal approaches in regenerative medicine ( Acute multidrug delivery via a wearable bioreactor facilitates long-term limb regeneration and functional recovery in adult Xenopus laevis - PMC ) ( Acute multidrug delivery via a wearable bioreactor facilitates long-term limb regeneration and functional recovery in adult Xenopus laevis - PMC ).
Top-down control in biology means manipulating higher-level variables (like tissue-level states or organ-level properties) to achieve an effect, rather than tweaking one gene at a time. Our conventional approaches (CRISPR gene editing, single-pathway drugs) are largely bottom-up. Top-down approaches might look like: using a focused electrical field to induce an organizer region in tissue, or using light to set a desired bioelectric pattern in a whole field of cells (optogenetic holography, say), which then causes genes to follow suit. The benefit of top-down methods is that they inherently engage the collective mechanisms – it’s like encouraging the cells to use their native programs to do the work, rather than brute-forcing them. The frog BioDome experiment is a taste of this: by providing a general “go regenerate” signal environment, it let the system self-organize a limb ( Acute multidrug delivery via a wearable bioreactor facilitates long-term limb regeneration and functional recovery in adult Xenopus laevis - PMC ). Another potential future tool is the idea of morphogenetic circuits – engineered cells that could be introduced to a tissue to locally impose a pattern (imagine a patch that “paints” a certain voltage on a wound to guide healing). All of these fall under leveraging the intrinsic intelligence of tissues: rather than building structures cell by cell (like tissue engineering currently often does), one could convince the cells to build the structure themselves by communicating in their own language.
With such capabilities, the possibilities are expansive. In regenerative medicine, we could address birth defects not by surgery but by correcting the developmental signals – for instance, fixing a craniofacial abnormality by re-establishing the correct bioelectric face prepattern in utero. In aging research, perhaps aging tissues lose some of their pattern memory; a “reboot” of their youthful bioelectric state might restore better structure and function. In synthetic biology, anatomical compilers could create organisms to order (with obvious ethical considerations) or grow complex bio-machines for tasks (e.g., an entity that eats plastic pollution, designed with a certain shape and behavior from the ground up).
Current Open Questions:
Despite the excitement, many known unknowns remain. We can enumerate a few key ones:
Mapping the Bioelectric Code:
We know only a handful of mappings (voltage pattern X leads to outcome Y). Systematically mapping which bioelectric states correspond to which anatomical outcomes is a huge task. It likely won’t be one-to-one (since context matters), but building a library of instructive patterns is an active goal ( The bioelectric code: An ancient computational medium for dynamic control of growth and form - PMC ). For now, much is done by trial and error, guided by hypotheses; a future “codebook” would greatly advance predictive control.
Mechanism of Interpretation:
How do cells read bioelectric patterns? The downstream pathways (e.g., voltage-gated calcium signaling, changes in pH, etc.) that translate an electrical state into gene expression changes are still being elucidated (Frequently Asked Questions - Latest Version). We have some clues (like voltage-regulated transporters affecting biochemical gradients, or bioelectric state influencing where transcription factors go), but a general theory is lacking.
Stability and Memory:
What gives bioelectric states their long-term stability? Is it purely ionic feedback loops, or do they get “hardwired” into tissue properties (like by changing ECM or cytoskeleton)? Levin mentions possible long-term storage in cytoskeletal or transcriptional states that maintain an electrical bias (Frequently Asked Questions - Latest Version). Understanding this could help us know how permanent or flexible a given pattern is, and how easily it can be re-written.
Interplay with Other Layers:
Bioelectricity doesn’t act alone; it interweaves with biochemical gradients and gene networks. We need integrative models that show how, say, a morphogen gradient and a bioelectric gradient cooperate or conflict. Some models propose that bioelectric patterns might set boundaries for biochemical patterns (electrical fields could corral where certain proteins accumulate). This multiscale integration is complex and currently a frontier.
Species Differences:
Not all species use bioelectric cues equally. For example, planaria and amphibians show dramatic bioelectric roles; what about mammals? There is evidence that bioelectricity influences mammalian regeneration (e.g., electric stimulation helps healing), but mammalian development occurs in utero with possibly more constraints. Understanding how universal these principles are is important if we are to translate to human medicine.
Ethical and Safety Concerns:
As we gain power to rewrite anatomical patterns, we face questions of unintended consequences. Could inducing a regeneration inadvertently cause a tumor? Could creating new lifeforms (xenobot-like) have ecological or ethical implications? Guiding complex systems top-down is tricky; we will need computational assistance (AI) to search safe intervention strategies and robust safeguards.
Unknown Unknowns:
Perhaps the most intriguing aspect is that this field likely hides “unknown unknowns” – phenomena we haven’t even conceptualized yet. For instance, could there be other signal modalities (besides chemical, mechanical, electrical) that cells use in morphogenesis, yet to be discovered? Some have speculated about bioacoustic signals or even electromagnetic fields beyond direct current flows. Or there may be deep links between developmental bioelectricity and brain function that could lead to insights about bioelectric aspects of memory and information storage in general ( The bioelectric code: An ancient computational medium for dynamic control of growth and form - PMC ). As we push the boundary, we may stumble on totally new principles of self-organization that not only advance biology but perhaps physics or computer science analogies as well.
Conclusion
The emerging picture of human (and animal) morphogenesis is one of a multi-layered control system combining genetic instructions, biochemical signals, mechanical forces, and bioelectrical networks. Traditional developmental biology provided the parts list and local rules – genes and proteins that cells use to differentiate and respond to neighbors. The radical new insights from Michael Levin’s research add the idea that there is a higher-level logic to how cells cooperate, implemented through bioelectric signaling that enables tissues to make decisions as a collective. DNA encodes the micro-level hardware, but the “software” of anatomy is encoded in physiological circuits and feedback loops (Frequently Asked Questions - Latest Version). This software allows cells to pursue large-scale goals like regenerating a limb or maintaining a body plan, acting in some ways like a distributed computing system. Morphogenesis, therefore, is not a rigid unfolding of a genetic program but a dance of genomic, electrical, and mechanical interactions – a dance that is choreographed by evolution yet improvised by cells in each individual.
We compared the roles of bioelectricity and genetics not to pit one against the other, but to show their interplay: where genetics provides a bottom-up foundation, bioelectricity enables top-down feedback and dynamic control. The case studies illustrate these principles in action: limb regeneration leverages the body’s modular memory of form, cancer results from a breakdown of cellular communication and can potentially be tamed by restoring that communication ( The bioelectric code: An ancient computational medium for dynamic control of growth and form - PMC ), and xenobots reveal the latent creative potential when cells are guided into new coalitions. These examples push us to broaden our notion of what cells are capable of.
At a theoretical level, this synthesis touches on deep questions: the nature of biological information, how evolution creates complexity, and how intelligence might originate from simple units. It hints that what we experience as our mind could be just one instance of a more general phenomenon of problem-solving in nature – our neurons’ chatter may be a specialized cousin of the chatter among embryonic cells assembling an organism (Bioelectric networks: the cognitive glue enabling evolutionary scaling from physiology to mind | Animal Cognition ). If so, understanding one can illuminate the other.
Finally, we looked ahead to the tantalizing possibility of directing morphogenesis at will – a dream that would have huge implications. Imagine being able to regenerate injured organs, reprogram tumors into normal tissue, or even grow tailor-made biological machines. The concept of an anatomical compiler is a beacon on the horizon, inspiring researchers to decode enough of the bioelectric and developmental code to make such control feasible. Achieving that will require embracing a top-down mindset in parallel with the bottom-up: recognizing system-level order as a cause in itself, not just an effect (Top-down models in biology: explanation and control of complex living systems above the molecular level | Journal of The Royal Society Interface) (Top-down models in biology: explanation and control of complex living systems above the molecular level | Journal of The Royal Society Interface).
In conclusion, the integration of classical developmental biology with Levin’s bioelectric and cognitive paradigm yields a far richer understanding of morphogenesis. It reveals life’s architecture as not only chemically and genetically driven, but also electrically communicated and computationally achieved. The knowns are that cells use more than genes to build bodies – they use volts and ions, they remember shapes, they have rudimentary goals. The known unknowns include deciphering the full bioelectric code and learning to manipulate it safely. And the unknown unknowns beckon us to explore boldly, as we stand on the cusp of a new synthesis of biology, one that treats geometry, information, and physiology as equal partners in the story of life’s form. As we continue to crack these codes, we move closer to not just understanding life’s incredible morphogenetic abilities, but actively harnessing them – a step that could transform medicine and our relationship with living systems in profound ways.
References
This thesis has drawn upon foundational concepts in developmental biology and key contributions from Michael Levin’s work and others in the field of bioelectricity and regenerative biology. Notable references include Levin’s comprehensive reviews on the bioelectric code and pattern memory ( The bioelectric code: An ancient computational medium for dynamic control of growth and form - PMC ) ( The bioelectric code: An ancient computational medium for dynamic control of growth and form - PMC ) (Scientists Grow Flatworm With Two Heads Instead Of Tail | IFLScience), empirical studies on limb regeneration and bioelectric induction ( Acute multidrug delivery via a wearable bioreactor facilitates long-term limb regeneration and functional recovery in adult Xenopus laevis - PMC ), insights into cancer as a developmental disorder ( The bioelectric code: An ancient computational medium for dynamic control of growth and form - PMC ), and descriptions of novel organisms like Xenobots (Scientists Assemble Frog Stem Cells Into First 'Living Machines'). These sources, among others cited throughout the text, provide evidence and context for the integrated perspective presented here. Together, they reflect an evolving scientific narrative: one that increasingly views cells as cognitive agents of morphology and bioelectric networks as the language by which they coordinate the symphony of development.
AI Reasoining
Towards a Unified Theory of Human MorphogenesisChatGPT
I’m thinking through how to merge traditional morphogenetic biology with Michael Levin’s insights. This involves covering classic processes, Levin’s bioelectric code, and case studies on limb regeneration, cancer, and synthetic lifeforms like Xenobots.
I’m organizing the thesis by outlining sections like Classical Morphogenesis, Michael Levin’s Contributions, Case Studies, and Theoretical Implications. This helps in systematically integrating ideas.
Searched for Michael Levin bioelectric cognitionChatGPT
I identified ScienceDirect as likely [0], a PMC link for Michael Levin's article [1], a Leving넔er bioscience medium [2], an Allen Institute blog [3], a PMC link for "Addendum" [4], a Frontiers paper [5], a TED talk [6], a Wyss Institute interview [7], a collective intelligence piece [8], and a SciDirect link [9].
Read pmc.ncbi.nlm.nih.gov
ChatGPT
I’m matching Levin's bioelectric insights with genetic explanations, filled with experiments highlighting cells' intentional and robust behavior. Molecular pathway experiments, like activating oncogenes, underscore this complex interplay.
I’m thinking about Xenobots, these frog embryonic cells organized into novel life forms. Their ability to show self-replication and morphological adaptability hints at bioelectricity's deep role in directing cellular behavior and form.
Searched for Michael Levin anatomical controlChatGPT
I’m thinking about Levin or his team, considering royalsocietypublishing and something like a Philosophical Transactions article. This aligns with the discussed topic.
Read royalsocietypublishing.org
ChatGPT
I'm gathering insights on a conceptual piece, potentially co-authored by Levin or a collaborator, discussing diverse molecular mechanisms achieving a common goal and implementation independence.
Read more from royalsocietypublishing.org
ChatGPT
I’m mapping out Michael Levin's perspective on bioelectric signaling, noting its influence on morphogenesis, and regeneration insights.
Piecing together insights on bioelectric signaling, classifying biological implications, and gauging future outlooks to understand form and regeneration.
Searched for developmental biology and morphogenesisChatGPT
I'm gathering general classical concepts and considering a quote or summary to enhance credibility, like a Wikipedia excerpt on morphogenesis.
Read en.wikipedia.org
ChatGPT
I’m exploring classical sources to confirm genes as development tools, morphogens, and tissue mechanics. Prioritizing peer-reviewed research and open-access texts for citations.
Read pmc.ncbi.nlm.nih.gov
ChatGPT
DNA encodes proteins but doesn't determine anatomical structures. Pattern control involves a code beyond DNA.
Read more from pmc.ncbi.nlm.nih.gov
ChatGPT
I’m mapping out key lab references on Xenopus tail regeneration, planarian head regeneration, and left-right asymmetry. Balancing progress with thoroughness should ensure clarity.
Read drmichaellevin.org
ChatGPT
Bioelectricity, a medium for cellular decisions, traces its roots to ancient electrical networks. Evolution favored it over brain computation for morphogenesis.
Read more from drmichaellevin.org
ChatGPT
Michael Levin's bioelectric code storage ideas suggest bioelectric signals drive tissue pattern formation, similar to brain memory systems. Fascinating three code modes emerge: one-to-one, hidden, and binary triggers. It’s intriguing to see how these concepts could revolutionize biomedical research.
Searched for Levin frog limb regeneration 2022ChatGPT
I’m weighing Science Advances and Science as plausible sources, noting the "www.science.org" reference. Considering NCBI for potential content.
Read pmc.ncbi.nlm.nih.gov
ChatGPT
After 24 hours of treatment and 18 months, frog limbs regrow with skin, bone, vessels, and nerves, supporting the claim of partial regeneration.
Read more from pmc.ncbi.nlm.nih.gov
ChatGPT
It's worth noting that the abstract emphasizes tissue regeneration using electrochemical and biomaterial stimuli, drawing on examples like salamanders and planaria, and mentioning a bioelectric code for references.
Read more from pmc.ncbi.nlm.nih.gov
ChatGPT
I’m curious about how planaria and axolotls regenerate their morphology, showing stability and the ability to maintain "target morphology" influenced by bioelectric states.
Read more from pmc.ncbi.nlm.nih.gov
ChatGPT
I'm linking the disruption of bioelectric signaling to cancer metastasis. Analyzing how cancer represents cells deviating from body cues and examining the concept of patterning. Progressing towards incorporating xenobot references.
Searched for Xenobots frog cells self-assemble 2020 PNAS
Read pnas.org
ChatGPT
Thinking through the necessity of signing in or verifying access to the PNAS site based on specific search results or article references.
Read more from pnas.org
ChatGPT
I'm thinking through other options like "Smithsonianmag" or "ScienceDaily" to find details about Xenobot since the direct PNAS site isn't providing the content.
Read smithsonianmag.com
Read link.springer.com
ChatGPT
I'm exploring how intelligence scales from cellular cooperation to larger systems, using bioelectric signaling principles. This includes morphogenesis, cancer suppression, and significant links to classical behavior.
Read more from link.springer.com
ChatGPT
I’m planning to structure the thesis with sections like Introduction, Classical Morphogenetic Mechanisms, Emerging Perspectives, Bioelectricity vs Genetic Determinism, Case Studies, Implications for the Origins of Form, Morphogenesis as Collective Intelligence, Future Directions, and Conclusions, ensuring logical progression and clarity.
Searched for Waddington epigenetic landscapeChatGPT
I’m mapping out the concept's specifics for clarity and precision.
Read pmc.ncbi.nlm.nih.gov
ChatGPT
Introducing bioelectric signaling as a new epigenetic process influencing patterning, Levin explores the encoded pattern memory's impact on tissue dynamics and applies insights to human morphogenesis, emphasizing human relevance in regeneration and birth defects.
Searched for Michael Levin anatomical compiler interviewChatGPT
I'm exploring how "The New Yorker" might use unique descriptions like "cancer as disobedient cells" to explain complex concepts to journalists. This approach adds depth to understanding.
Read thoughtforms.life
ChatGPT
I'm pulling together references to ensure academic integrity, avoiding playful elements while citing reputable sources.
Reframing speculative ideas
I’m considering how speculative anatomical compilers could be reframed from controversial top-down approaches to more scientifically grounded concepts. Progress includes defining top-down causes and linking ion channels to physiological software.
Read th-thumbnailer.cdn-si-edu.com
ChatGPT
I'm thinking of embedding an 800x600 image of a xenobot at the beginning of the Xenobot case study section. Also, considering images of regeneration or bioelectric maps if available.
Searched for Levin two-headed planarian experiment
Read iflscience.com
ChatGPT
Pulling together citations to create a coherent, logically flowing final answer, ensuring careful paraphrasing and incorporation of sources.
Read drmichaellevin.org
ChatGPT
I'm gathering the essential references to craft a thorough final answer.
Sources
Citationspmc.ncbi.nlm.nih.govThe bioelectric code: An ancient computational medium for dynamic control of growth and form - PMCWhat determines large-scale anatomy? DNA does not directly specify geometrical arrangements of tissues and organs, and a process of encoding and decoding for morphogenesis is required. Moreover, many species can regenerate and remodel their structure despite drastic injury. The ability to obtain the correct targetpmc.ncbi.nlm.nih.govThe bioelectric code: An ancient computational medium for dynamic control of growth and form - PMCAlongside the genetic and (chromatin) epigenetic codes operates a crucial set of controls called the bioelectric code. While the encoding of pattern outcomes to bioelectric states is only known for a small handful of examples, it is clear that profound lessons about the source and nature of information that determinespmc.ncbi.nlm.nih.govThe bioelectric code: An ancient computational medium for dynamic control of growth and form - PMCmorphogenesis is required. Moreover, many species can regenerate and remodel their structure despite drastic injury. The ability to obtain the correct target morphology from a diversity of initial conditions reveals that the morphogenetic code implements a rich system of pattern-homeostatic processes.drmichaellevin.orgFrequently Asked Questions - Latest VersionBioelectricity refers to signals carried by the voltage gradients, ion flows, and electric fields that all cells receive and emit. It has been known for over 100 years that all cells, not just excitable nerve and muscle, exhibit steady- state long-term bioelectrical activity, and that this activity appears to be instructive for cell behavior during morphogenesis. While bioelectricity functions alongside biochemical and biomechanical events, it has a unique aspect. Much like in neuroscience, bioelectricity is the computational medium with which cellular collectives make decisions (about growth and form). Evolution discovered that electrical networks are a great way to compute, long before brains and muscle came on the scene. Bioelectricity is an ancientiflscience.comScientists Grow Flatworm With Two Heads Instead Of Tail | IFLScience“With this work, we now know that bioelectric properties can permanently override the default body shape called for by a genome, that regenerative target morphology can be edited to diverge from the current anatomy, and that bioelectric networks can be a control point for investigating cryptic,pmc.ncbi.nlm.nih.govThe bioelectric code: An ancient computational medium for dynamic control of growth and form - PMC2009), while axolotls can regenerate eyes, limbs, tails, jaws, ovaries, and portions of the brain (Maden, 2008). Such examples reveal that living systems exhibit highly adaptive and context-sensitive pattern homeostasis. Individual cell behaviors are directed towards the maintenance and repair of aen.wikipedia.orgMorphogenesis - WikipediaImageMorphogenesis is controlled by a "toolkit" of genes which switch development on and off at precise times and places. Here, gap genes in the fruit fly are switched on by genes such as bicoid, setting up stripes which create the body's segmental form.en.wikipedia.orgMorphogenesis - WikipediaSeveral types of molecules are important in morphogenesis. Morphogens are soluble molecules that can diffuse and carry signals that control cell differentiation via concentration gradients. Morphogens typically act through binding to specific protein receptors. An important class of moleculespmc.ncbi.nlm.nih.govThe bioelectric code: An ancient computational medium for dynamic control of growth and form - PMC2006), and epigenetic codes (Broccoli et al., 2015) that regulate transcriptional cascades via chromatin modification. However, the processes underlying embryogenesis are largely thought of as a ‘feed-forward’ system: the progressive unrolling of the genome in each cell results in specific cellularen.wikipedia.orgMorphogenesis - Wikipediamatch at L226 Several types of molecules are important in morphogenesis. Morphogens are soluble molecules that can diffuse and carry signals that control cell differentiation via concentration gradients. Morphogens typically act through binding to specific protein receptors. An important class of moleculesen.wikipedia.orgMorphogenesis - Wikipediamorphogenesis include Wnt, Hedgehog, and ephrins.[ 8 ]en.wikipedia.orgMorphogenesis - WikipediaAt a tissue level, ignoring the means of control, morphogenesis arises because of cellular proliferation and motility.[ 9 ] Morphogenesis also involves changes in the cellular structure[ 10 ] or how cells interact in tissues. These changes can result in tissue elongation, thinning, folding, invasion or separation offrontiersin.orgEmerging Role of Mechanical Forces in Cell Fate AcquisitionAcquisition www.frontiersin.org Mechanical forces are now recognized as central players in tissue morphogenesis that drive changes in cell shape, size, proliferation, and movement.en.wikipedia.orgMorphogenesis - Wikipediapatterns such as the spirals of 111, were written by D'Arcy Wentworth Thompson in his 1917 book 113[ 2 ][ 3 ][ note 1 ] and Alan Turing in his 115 (1952).[ 6 ] Where Thompson explained animal body shapes as being created by varying rates of growth in different directions, for instance to create the spiral shell of a 117, Turing correctly predicted a mechanism of morphogenesis, the diffusion of two different chemical signals, one activating and one deactivating growth, to set up patterns of development, decades before the formation of such patterns was observed.[ 7 ] The fullerdrmichaellevin.orgFrequently Asked Questions - Latest Versioninstructive for cell behavior during morphogenesis. While bioelectricity functions alongside biochemical and biomechanical events, it has a unique aspect. Much like in neuroscience, bioelectricity is the computational medium with which cellular collectives make decisions (about growth and form). Evolution discovered that electrical networks are a great way to compute, long before brains and muscle came on the scene. Bioelectricity is an ancient modality that serves as the proto-cognitive medium of the cellular collective intelligence that navigates morphospace (the space of possible anatomies). As such, it is a powerful interface that cells and tissues expose to us (and todrmichaellevin.orgFrequently Asked Questions - Latest Versionsuch as cytoskeleton structures, etc.). The notion that "body pattern is stored in the DNA" is not that simple, depending on what you're asking. What is stored in the DNA is protein sequences, which is single cell-level hardware information. Bioelectric patterns emerge from the complex dynamical interactions of ion channels and gap junctions opening and closing, and it's that physiological software that stores and processes patterning information. 3. deeper still: There needs to be agreement on what "storing a code" really means. It's not simple, and there's a lot of work on this. Things are only codes with respect to what is reading or interpreting the code. So what we reallydrmichaellevin.orgFrequently Asked Questions - Latest Versioneasy to store encodings in electrical states of a medium that holds patterns over long time. All tissues - not just brains - do that. So, the excitable medium which can store information is the voltage state of groups of cells (another more familiar medium is DNA in groups of cells, and there are others such as cytoskeleton structures, etc.). The notion that "body pattern is stored in the DNA" is not that simple, depending on what you're asking. What is stored in the DNA is protein sequences, which is single cell-level hardware information. Bioelectric patterns emerge from the complex dynamical interactions of ion channels and gap junctions opening and closing, and it's thatpmc.ncbi.nlm.nih.govThe bioelectric code: An ancient computational medium for dynamic control of growth and form - PMCanatomy. Bioelectric signaling is a new type of epigenetic process, with dynamics quite distinct from that of chromatin-modifying effects as it is fundamentally distributed (multi-cellular scale) and encodes patterning, not only metabolic and differentiation state information.pmc.ncbi.nlm.nih.govThe bioelectric code: An ancient computational medium for dynamic control of growth and form - PMCnext consider the role of bioelectrical events in encoding and decoding anatomical structure, keeping in mind that bioelectrics is perhaps but a single component of the rich morphogenetic code that regulates the shape of life at all scales.pmc.ncbi.nlm.nih.govThe bioelectric code: An ancient computational medium for dynamic control of growth and form - PMCthe case of tissues) from bioelectrical state readings. The computational analogy is not meant to suggest that tissues (or the brain) operate specifically via the Von Neumann architecture used by today’s computers. Interestingly, the CNS provides important input into pattern regulation. When a nerve cord is cutpmc.ncbi.nlm.nih.govThe bioelectric code: An ancient computational medium for dynamic control of growth and form - PMCmatch at L846 produced in this way continue to regenerate as double-headed when the ectopic heads are amputated, in plain water, revealing that brief (48 h) targeting of the bioelectric network to induce a different circuit state (with depolarized regions at both ends) permanently re-species the target morphology to which eachiflscience.comScientists Grow Flatworm With Two Heads Instead Of Tail | IFLScienceby rewiring its bioelectricity.pmc.ncbi.nlm.nih.govThe bioelectric code: An ancient computational medium for dynamic control of growth and form - PMCproduced in this way continue to regenerate as double-headed when the ectopic heads are amputated, in plain water, revealing that brief (48 h) targeting of the bioelectric network to induce a different circuit state (with depolarized regions at both ends) permanently re-species the target morphology to which eachpmc.ncbi.nlm.nih.govThe bioelectric code: An ancient computational medium for dynamic control of growth and form - PMCproduced in this way continue to regenerate as double-headed when the ectopic heads are amputated, in plain water, revealing that brief (48 h) targeting of the bioelectric network to induce a different circuit state (with depolarized regions at both ends) permanently re-species the target morphology to which eachpmc.ncbi.nlm.nih.govThe bioelectric code: An ancient computational medium for dynamic control of growth and form - PMCmatch at L807 piece of Girardia dorotocephala planarian to regenerate heads appropriate to two other species of planaria. Fragments of Girardia flatworms, treated with a gap junction uncoupler, regenerated head shapes, brain morphology, and stem cell distributions appropriate to two other extant species of planaria andlink.springer.comBioelectric networks: the cognitive glue enabling evolutionary scaling from physiology to mind | Animal Cognitiondistinct from the emergent dynamics of termite mounds and other swarms, the reality is that all intelligence is collective intelligence: each of us consists of a huge number of cells working together to generate a coherent cognitive being with goals, preferences, and memories that belong to the whole and not to its parts. Basal cognition is the quest to understand how Mind scales—how large numbers of competent subunits can work together to become intelligences that expand the scale of their possible goals. Crucially, the remarkable trick of turning homeostatic, cell-level physiological competencies into large-scale behavioral intelligences is not limited to the electrical dynamics of the brain.drmichaellevin.orgFrequently Asked Questions - Latest VersionBioelectricity refers to signals carried by the voltage gradients, ion flows, and electric fields that all cells receive and emit. It has been known for over 100 years that all cells, not just excitable nerve and muscle, exhibit steady- state long-term bioelectrical activity, and that this activity appears to be instructive for cell behavior during morphogenesis. While bioelectricity functions alongside biochemical and biomechanical events, it has a unique aspect. Much like in neuroscience, bioelectricity is the computational medium with which cellular collectives make decisions (about growth and form). Evolution discovered that electrical networks are a great way to compute, long before brains and muscle came on the scene. Bioelectricity is an ancientdrmichaellevin.orgFrequently Asked Questions - Latest Versionaspect. Much like in neuroscience, bioelectricity is the computational medium with which cellular collectives make decisions (about growth and form). Evolution discovered that electrical networks are a great way to compute, long before brains and muscle came on the scene. Bioelectricity is an ancient modality that serves as the proto-cognitive medium of the cellular collective intelligence that navigates morphospace (the space of possible anatomies). As such, it is a powerful interface that cells and tissues expose to us (and to each other) that enable reprogramming for biomedical purposes (and for understanding evolutionary change).pmc.ncbi.nlm.nih.govThe bioelectric code: An ancient computational medium for dynamic control of growth and form - PMCgiving rise to perfectly normal monozygotic twins each of which has regenerated the missing cell mass. (D) Their embryos can also be combined, giving rise to a normal (but slightly larger) embryo in which no parts are duplicated. (E) The ability to achieve a specific target morphology despite different startingdrmichaellevin.orgFrequently Asked Questions - Latest Versionaspect. Much like in neuroscience, bioelectricity is the computational medium with which cellular collectives make decisions (about growth and form). Evolution discovered that electrical networks are a great way to compute, long before brains and muscle came on the scene. Bioelectricity is an ancient modality that serves as the proto-cognitive medium of the cellular collective intelligence that navigates morphospace (the space of possible anatomies). As such, it is a powerful interface that cells and tissues expose to us (and to each other) that enable reprogramming for biomedical purposes (and for understanding evolutionary change).link.springer.comBioelectric networks: the cognitive glue enabling evolutionary scaling from physiology to mind | Animal Cognitionappeared, to solve the problem of creating and repairing complex bodies. In this Perspective, I review the deep symmetry between the intelligence of developmental morphogenesis and that of classical behavior. I describe the highly conserved mechanisms that enable the collective intelligence of cells to implement regulative embryogenesis, regeneration, and cancer suppression. I sketch the story of an evolutionary pivot that repurposed the algorithms and cellular machinery that enable navigation of morphospace into the behavioral navigation of the 3D world which we so readily recognize as intelligence. Understanding the bioelectric dynamics that underlie construction of complexpmc.ncbi.nlm.nih.govThe bioelectric code: An ancient computational medium for dynamic control of growth and form - PMClies within the genome, but it is not that simple; DNA simply encodes specific proteins – there is no direct encoding of anatomical structure. Thus, it is clear from first principles that pattern control involves a code: the encoding of anatomical positions and structures within the egg or other cell type, anddrmichaellevin.orgFrequently Asked Questions - Latest Versionstate of the art now - interpretation - which we still poorly understand but are working on. And lest we get too comfortable with how well the "DNA code" has been decoded, let's remember that we have no ability to predict anatomy from genome (other than by cheating - comparison with known genomes) and we can't tell in advance if a frogolotl (mixed embryo of frog and axolotl cells) will have legs, by having frog and axolotl genomes.royalsocietypublishing.orgTop-down models in biology: explanation and control of complex living systems above the molecular level | Journal of The Royal Society Interfaceevents) is unnecessarily limiting. It is not known whether bottom-up strategies can optimally explain large-scale properties such as self-repairing anatomy, or whether they best facilitate interventions strategies for rationally altering systems-level properties. However, it has been observed that a number of biological systems seem to use highly diverse underlying molecular mechanisms to reach the same high-level (e.g. topological) goal state (figure 1 c), suggesting a kind of ‘implementation independence’ principle that focuses attention on the global state as a homeostatic target for cellular and molecular activities.royalsocietypublishing.orgTop-down models in biology: explanation and control of complex living systems above the molecular level | Journal of The Royal Society Interfacebiological systems seem to use highly diverse underlying molecular mechanisms to reach the same high-level (e.g. topological) goal state (figure 1 c), suggesting a kind of ‘implementation independence’ principle that focuses attention on the global state as a homeostatic target for cellular and molecular activities.royalsocietypublishing.orgTop-down models in biology: explanation and control of complex living systems above the molecular level | Journal of The Royal Society InterfaceMany biological functions, such as pattern homeostasis, are readily viewed in terms of systems that seek to acquire and maintain specific large-scale states. A central example is dynamic anatomical re-configuration; pattern homeostasis, as seen in highly regenerative animals, is difficult to explain or control via molecular-level models. This is because the global control metrics (e.g. ‘numberpmc.ncbi.nlm.nih.govThe bioelectric code: An ancient computational medium for dynamic control of growth and form - PMCmutations and yet their morphology is robust – they regenerate a correct planarian anatomy every single time, without developing cancer or aging. Moreover, planaria are the only model system in which no genetic patterning mutant lines are available. Every other model (C. elegans, zebrafish, chick,pmc.ncbi.nlm.nih.govThe bioelectric code: An ancient computational medium for dynamic control of growth and form - PMC5. Adams DS, Masi A, Levin M, 2007. H+ pump-dependent changes in membrane voltage are an early mechanism necessary and sufficient to induce Xenopus tail regeneration. Development 134, 1323–1335. [DOI] [PubMed] [Google Scholar]pmc.ncbi.nlm.nih.govThe bioelectric code: An ancient computational medium for dynamic control of growth and form - PMCmatch at L1238 6. Adams DS, Tseng AS, Levin M, 2013. Light-activation of the Archaerhodopsin H(+)-pump reverses age-dependent loss of vertebrate regeneration sparking system-level controls in vivo. Biol. Open 2, 306–313. [DOI] [PMC free article] [PubMed] [Googledrmichaellevin.orgFrequently Asked Questions - Latest Versiontissues. There are 3 basic modes we've found: a) 1:1 prepatterns (like the electric face or your brain pattern), b) non-1:1 prepatterns encoding specific organs, like planarian head-tail info (which can be mapped onto heads or tails but it is not visually obvious like for electric face) or eye spots, or c) binary triggers that say "build whatever goes here" like the tail/leg signals (which carry almost none of the detailed info of how to build it). This is the state of the art now - interpretation - which we still poorly understand but are working on. And lest we get too comfortable with how well the "DNA code" haspmc.ncbi.nlm.nih.govAcute multidrug delivery via a wearable bioreactor facilitates long-term limb regeneration and functional recovery in adult Xenopus laevis - PMClong-term (18 months) regrowth, marked tissue repatterning, and functional restoration of an amputated X. laevis hindlimb following a 24-hour exposure to a multidrug, pro-regenerative treatment delivered by a wearable bioreactor. Regenerated tissues composed of skin, bone, vasculature, and nervespmc.ncbi.nlm.nih.govAcute multidrug delivery via a wearable bioreactor facilitates long-term limb regeneration and functional recovery in adult Xenopus laevis - PMCto test interventions that can restore form and function. Here, we demonstrate long-term (18 months) regrowth, marked tissue repatterning, and functional restoration of an amputated X. laevis hindlimb following a 24-hour exposure to a multidrug, pro-regenerative treatment delivered by a wearable bioreactor. Regenerated tissues composed of skin, bone, vasculature, and nerves significantly exceeded the complexity and sensorimotor capacities of untreated and control animals’ hypomorphic spikes. RNA sequencing of early tissue buds revealed activation of developmental pathways such as Wnt/β-catenin, TGF-β, hedgehog, and Notch. These data demonstrate the successful “kickstarting” of endogenous regenerative pathways in a vertebrate model.pmc.ncbi.nlm.nih.govAcute multidrug delivery via a wearable bioreactor facilitates long-term limb regeneration and functional recovery in adult Xenopus laevis - PMCRegenerated tissues composed of skin, bone, vasculature, and nerves significantly exceeded the complexity and sensorimotor capacities of untreated and control animals’ hypomorphic spikes. RNA sequencing of early tissue buds revealed activation of developmental pathways such as Wnt/β-catenin, TGF-β, hedgehog, and Notch. These data demonstrate the successful “kickstarting” of endogenous regenerative pathways in a vertebrate model.pmc.ncbi.nlm.nih.govAcute multidrug delivery via a wearable bioreactor facilitates long-term limb regeneration and functional recovery in adult Xenopus laevis - PMCcues that organize cells into heterogeneous, intricately patterned, three- dimensional (3D) structures. Thus, an important new direction for the field of regenerative medicine is the discovery of interventions that trigger latent developmental or embryogenic cascades (17 , 39), shifting the burden of morphogenetic control onto the cells and tissues themselves. Combinations of electrochemical and biomaterial-based treatments would further overcome previous pitfalls by offering both information-rich signals and structural stability.iflscience.comScientists Grow Flatworm With Two Heads Instead Of Tail | IFLScienceThis led the team to conclude that bioelectrical patterns can determine what the flatworms will become after they've regenerated – rather than stem cells.iflscience.comScientists Grow Flatworm With Two Heads Instead Of Tail | IFLSciencematch at L235 This led the team to conclude that bioelectrical patterns can determine what the flatworms will become after they've regenerated – rather than stem cells.pmc.ncbi.nlm.nih.govThe bioelectric code: An ancient computational medium for dynamic control of growth and form - PMCwork showing that, contrary to the view of cancer as exclusively an irrevocable phenotype caused by clonal expansion of genetically mutated founder cells, metastatic disease can be triggered by disruption of bioelectric signaling (Blackiston et al., 2011; Lobikin et al., 2015; Morokuma etpmc.ncbi.nlm.nih.govThe bioelectric code: An ancient computational medium for dynamic control of growth and form - PMCto spec, on demand. Even cancer, the defection of cells from the body’s patterning cues, could be addressed via reprograming (normalization), as could the creation of arbitrarily-complex artificial constructs in vitro. Likewise, we cannot understand the evolutionary process until we really understand thelink.springer.comBioelectric networks: the cognitive glue enabling evolutionary scaling from physiology to mind | Animal Cognitionbioelectric state—normalizing cancer by reconnecting cells to the electrical network that harnesses them toward adaptive tissue homeostasis (Chernet et al. 2016, 2015; Chernet and Levin 2013b), a promising alternative to current toxic chemotherapy approaches.pmc.ncbi.nlm.nih.govThe bioelectric code: An ancient computational medium for dynamic control of growth and form - PMConcogene-induced tumorigenesis, and metastasis: the bioelectric basis of cancer in vivo. Phys. Biol 9, 065002. [DOI] [PMC free article] [PubMed] [Google Scholar] 116. Lobikin M, Lobo D, Blackiston DJ, Martyniuk CJ, Tkachenko E, Levin M,smithsonianmag.comScientists Assemble Frog Stem Cells Into First 'Living Machines'Levin and his colleagues have found that skin cells and heart cells can be coaxed into coalescing. Placed side by side, they will self-organize into intricate, three-dimensional mosaics of frog cells that aren’t actually frogs.smithsonianmag.comScientists Assemble Frog Stem Cells Into First 'Living Machines'Designed by a computer algorithm and surgically shaped by human hands, these skin-heart hybrids, each roughly the size of a grain of sand, don’t resemble anything found in nature. But the tasks they accomplish are eerily familiar: Without any external input, they can zoom around Petri dishes, push microscopic objects to and fro, and even stitch themselves back together after being cut.smithsonianmag.comScientists Assemble Frog Stem Cells Into First 'Living Machines'Image: xenobot 1 A "living machine" containing frog stem cells in a new configuration designed by a computer algorithm. Parts shown in green are made up of frog skin cells, while parts in red are frog heart cells. Kriegman et al., PNAS, 2020smithsonianmag.comScientists Assemble Frog Stem Cells Into First 'Living Machines'Designed by a computer algorithm and surgically shaped by human hands, these skin-heart hybrids, each roughly the size of a grain of sand, don’t resemble anything found in nature. But the tasks they accomplish are eerily familiar: Without any external input, they can zoom around Petri dishes, push microscopic objects to and fro, and even stitch themselves back together after being cut.smithsonianmag.comScientists Assemble Frog Stem Cells Into First 'Living Machines'Levin calls these clusters of cells a “new form of life”—one that’s not quite an organism and not quite a machine, but perhaps somewhere in between. Namedsmithsonianmag.comScientists Assemble Frog Stem Cells Into First 'Living Machines'In Michael Levin’s laboratory at Tufts University, cells can expect to find themselves in unusual company.pnas.orgKinematic self-replication in reconfigurable organisms - PNASA swarm of frog-cell parents push frog cells into piles that mature into self- moving “children”. An AI method optimizes the original ...smithsonianmag.comScientists Assemble Frog Stem Cells Into First 'Living Machines'Image: xenobot 1 A "living machine" containing frog stem cells in a new configuration designed by a computer algorithm. Parts shown in green are made up of frog skin cells, while parts in red are frog heart cells. Kriegman et al., PNAS, 2020link.springer.comBioelectric networks: the cognitive glue enabling evolutionary scaling from physiology to mind | Animal CognitionIn addition, even though we feel ourselves to be a unified, single Self, distinct from the emergent dynamics of termite mounds and other swarms, the reality is that all intelligence is collective intelligence: each of us consists of a huge number of cells working together to generate a coherent cognitive being with goals, preferences, and memories that belong to the whole and not to its parts. Basal cognition is the quest to understand how Mind scales—how large numbers of competent subunits can work together to become intelligences that expand the scale of their possible goals. Crucially, the remarkable trick of turning homeostatic, cell-level physiological competencies into large-scalelink.springer.comBioelectric networks: the cognitive glue enabling evolutionary scaling from physiology to mind | Animal Cognitionits parts. Basal cognition is the quest to understand how Mind scales—how large numbers of competent subunits can work together to become intelligences that expand the scale of their possible goals. Crucially, the remarkable trick of turning homeostatic, cell-level physiological competencies into large-scale behavioral intelligences is not limited to the electrical dynamics of the brain. Evolution was using bioelectric signaling long before neurons and muscles appeared, to solve the problem of creating and repairing complex bodies. In this Perspective, I review the deep symmetry between the intelligence of developmental morphogenesis and that of classical behavior. I describe thelink.springer.comBioelectric networks: the cognitive glue enabling evolutionary scaling from physiology to mind | Animal Cognitiondevelopmental morphogenesis and that of classical behavior. I describe the highly conserved mechanisms that enable the collective intelligence of cells to implement regulative embryogenesis, regeneration, and cancer suppression. I sketch the story of an evolutionary pivot that repurposed the algorithms and cellular machinery that enable navigation of morphospace into the behaviorallink.springer.comBioelectric networks: the cognitive glue enabling evolutionary scaling from physiology to mind | Animal Cognitiondevelopmental morphogenesis and that of classical behavior. I describe the highly conserved mechanisms that enable the collective intelligence of cells to implement regulative embryogenesis, regeneration, and cancer suppression. I sketch the story of an evolutionary pivot that repurposed the algorithms and cellular machinery that enable navigation of morphospace into the behavioral navigation of the 3D world which we so readily recognize as intelligence. Understanding the bioelectric dynamics that underlie construction of complexlink.springer.comBioelectric networks: the cognitive glue enabling evolutionary scaling from physiology to mind | Animal Cognitionregeneration, and cancer suppression. C This isomorphism between somatic and neural bioelectricity is what enables all of the tools of neuroscience to be used outside of the brain. Developmental bioelectricity is studied by voltage imaging dyes, and functional techniques such as genetic, chemical, and opticalthoughtforms.lifeForms of life, forms of mind | Dr. Michael Levin | Videos of, and transcripts of Q&A after, a few of my recent talksthese claims about intelligence and minds and so on are fundamentally interaction protocol claims. So, what you’re really saying is, yes, you can train it, or no, it doesn’t remember past the next five minutes, or yes, it can do certain specific things. I’ve stayed away from issues of first person consciousness and so on, not because it’s not important, but just because it’s hard enough to get people to think about these functional kinds of things in non-brainy agents, so I often stay within 3rd-person observable, really tight experimental kinds of tractable approaches. To the extent that we understand the types of intelligence that something can deploy, we can better make use of themthoughtforms.lifeForms of life, forms of mind | Dr. Michael Levin | Videos of, and transcripts of Q&A after, a few of my recent talksAnd I think because of the conservation of both bioelectric mechanisms and algorithms, for exactly the same reasons that we think our brains are home to consciousness, we should consider that some of our organs may have some of that too. And I don’t think it’s surprising at all that we don’t feel them. We don’t feel that with each other either – we have to infer other minds from experiments and interaction. And that’s exactly what I propose to do with all of these other unconventional systems.royalsocietypublishing.orgTop-down models in biology: explanation and control of complex living systems above the molecular level | Journal of The Royal Society Interfaceprocess models. One reason why top-down approaches have not been popular in modern biology (with exceptions, see below) is that they apparently embed a ‘dangerous’ notion of teleology, directedness, purpose or finalism. After all, evolutionary theory rejects the idea that processes are ‘directed’ towards someroyalsocietypublishing.orgTop-down models in biology: explanation and control of complex living systems above the molecular level | Journal of The Royal Society Interfacemodels. We argue that top-down control models provide a valuable complement to the toolkit of cell biologists, evolutionary biologists, bioengineers and workers in regenerative medicine because they provide a mechanistic roadmap for optimal explanation and control of some complex systems.royalsocietypublishing.orgTop-down models in biology: explanation and control of complex living systems above the molecular level | Journal of The Royal Society Interfacecurrent paradigm beyond emergence and molecule-level rules. We define top-down control in a biological context, discuss the examples of how cognitive neuroscience and physics exploit these strategies, and illustrate areas in which they may offer significant advantages as complements to the mainstreamroyalsocietypublishing.orgTop-down models in biology: explanation and control of complex living systems above the molecular level | Journal of The Royal Society Interface# 1.2. Top-down models: a complement to emergencethoughtforms.lifeForms of life, forms of mind | Dr. Michael Levin | Videos of, and transcripts of Q&A after, a few of my recent talksthere. That’s what I want from this anatomical compiler – you’ll sit down and say “I want a frog with six legs and wings and a propeller on top” and it should give the bioelectrical code messages to make the cells do that. Some people think that’s not possible, that there are developmental constraints where cellspmc.ncbi.nlm.nih.govAcute multidrug delivery via a wearable bioreactor facilitates long-term limb regeneration and functional recovery in adult Xenopus laevis - PMCPrevious efforts attempted to induce limb regeneration in nonregenerative models with the use of electrical stimulation (2 – 30), tissue-guiding biomaterials (7 ), progenitor cell transplantation ( 32–10 ), and the direct up-regulation of key molecular pathways ( 34, 12 ). However, all reports of experimentally stimulating regeneration have largely involved fully or partially regenerative model systems such as axolotls, anurans on the boundary of regeneration, or developmentally immature (i.e., nonadult) phenotypes ( 36–16). Limited success has been reported in restoring substantial growth and patterning of new functional limbs in nonregenerative adults. While previous approaches have focused on driving specific facets of thedrmichaellevin.orgFrequently Asked Questions - Latest Versionmeans. It's not simple, and there's a lot of work on this. Things are only codes with respect to what is reading or interpreting the code. So what we really need to do is talk about how bioelectric properties are interpreted by the tissues. There are 3 basic modes we've found: a) 1:1 prepatterns (like the electric face or your brain pattern), b) non-1:1 prepatterns encoding specific organs, like planarian head-tail info (which can be mapped onto heads or tails but it is not visually obvious like for electric face) or eye spots, or c) binary triggers that say "build whatever goes here" like the tail/leg signalsdrmichaellevin.orgFrequently Asked Questions - Latest Version1. simplest/shortest: it's stored in very much the same way as information in the brain: in the electric states of cells (just like in neurons) and downstream modifications (long-term storage in cytoskeletal and transcriptional states). 2. better: "it's stored in the stable bioelectric states maintained by cell networks." Just like in the brain, groups of cells make electrical networks that can stably store information. This is routinely modeled in neuroscience and is the basis of much of our technology; like memory circuits in volatile RAM, it's easy to store encodings in electrical states of a medium that holds patternspmc.ncbi.nlm.nih.govThe bioelectric code: An ancient computational medium for dynamic control of growth and form - PMCthose involved in development and cancer. Given the ancient origin of bioelectricity and the molecular conservation of mechanisms and algorithms by which the brain and body compute, how much overlap might there be between the developmental bioelectric code and the neural bioelectric code that underliesroyalsocietypublishing.orgTop-down models in biology: explanation and control of complex living systems above the molecular level | Journal of The Royal Society InterfaceHowever, top-down models, which have been very effectively exploited in sciences such as physics, computer science and computational neuroscience, present aroyalsocietypublishing.orgTop-down models in biology: explanation and control of complex living systems above the molecular level | Journal of The Royal Society Interfacematch at L411 models. We argue that top-down control models provide a valuable complement to the toolkit o